Post-Dispersal Seed Fate of Ocotea floribunda (Lauraceae) in Monteverde, Costa Rica
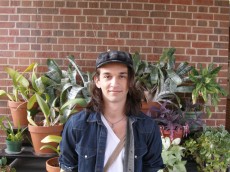
Luis Carlos Beltrán
Department of Biology
Lake Forest College
Lake Forest, IL 60045
Abstract
Ocotea floribunda (Lauraceae) is a Neotropical, bird-dispersed,
canopy tree species commonly found in Monteverde, Costa
Rica. The post-dispersal fate of seeds was studied to determine
how regurgitation by avian frugivores and microhabitat characteristics
affect seed removal through a Giving-Up Density (GUD) experiment.
The fate of removed seeds was assessed through a concurrent
seed-tagging experiment. An on-site camera trap revealed that agoutis
(Dasyprocta punctata) remove regurgitated seeds O. floribunda in
the open site. Microhabitat characteristics and seed treatment had
no significant effect on seed removal rates. Regurgitated seeds were
buried in the seed-tagging experiment. Seed infestation rates were
assessed following the termination of both experiments, which revealed
that arthropods infest more than two-thirds of all regurgitated
seeds. If, like O. endresiana, O. floribunda seeds do not successfully
germinate when buried (Wenny 2000), agoutis could be detrimental to
restoration efforts in Monteverde that focus on Lauraceous species.
Introduction
This thesis focuses on the post-dispersal seed fate of a Neotropical,
bird-dispersed, wild avocado species in Monteverde, Costa
Rica. I begin with three chapters that review foraging theory and seed
dispersal. The fourth chapter introduces the Lauraceae dispersal system
and places the experiments I carried out in context of the factors
relevant to post-dispersal seed fate. Chapters 5-7 present the methods
I followed for my experiments, the results, and discussion, respectively.
Chapter 1: Optimal Foraging Theory
Survival of a foraging animal is dependent on the forager’s ability
to find food, which it encounters as patches of variable resource richness.
As a forager depletes resources in a given patch, the richness of
the exploited patch will invariably decrease to a point where foraging is no
longer profitable (Fig. 1). Faced with this scenario, behavioral ecologists
seek to investigate how animals assess the resource richness of different
patches and decide how much to forage in a given patch. Proponents of
Optimal Foraging Theory (OFT) contend that an animal will continue to
forage from a given patch as long as the cost of engaging in a foraging action
does not exceed its gain (MacArthur and Pianka 1966, Royama 1971,
Krebs et al. 1974, Schoener 1974, Charnov 1976). It is most profitable
for foragers to search for patches with high levels of resource richness
(Royama 1970), but a forager’s inability to maximize feeding efficiency,
as well as the compromises made by foragers as a result of conflicting
selection pressures, will lead to sub-optimal foraging (Royama 1971).
Furthermore, opponents of OFT argue that the assumption that foragers
can instantaneously assess patch richness is flawed. Instead, foragers
are constantly learning about their environments in an attempt to forage
optimally, though optimal foraging is never achieved (Pierce and Ollasen
1987). While these so-called Bayesian foragers that assess patch richness
as they forage are indeed common (Green 1979), variations in foraging
strategies have been described, including prescient foragers with considerable
sensory capabilities that are capable of making unbiased estimates
of resource density, prior to patch exploitation (Valone and Brown 1989).
Thus, while not all animals forage optimally, a cost-benefit analysis of foraging
is still possible and can be applied to determine habitat preferences
(Stapanian and Smith 1984, Thorson et al. 1998, Jacob and Brown 2000,
Jansen and Forget 2001), food preferences and diet optimization
(Pulliam
1974, 1975, Steele et al. 1996), predation risk (Lima 1988, Lima and
Dill 1990, Thorson et al. 1998, Kotler et al. 2002), interspecific competition
(MacArthur and Levins 1964, Pianka 1969), and apparent competition
(Holt 1977), as long as alternative activities are incorporated into the
experiments and the model is appropriately modified (Brown 1988, Newman
1991). Animals do not have to hunt or forage optimally for insights
on foraging behavior, coexistence, and seed dispersal to be drawn using
OFT models (MacArthur and Levins 1964, Brown 1988, Newman 1991).
Figure 1. As a forager depletes resources from a patch, the foraging gain per unit of patch-residence time (Tp) decreases until foraging in that patch is no longer profitable. The point at which the foraging gain curve is closest to the dashed line represents the optimal time a forager should spend foraging at a patch. Tt represents travel time
OFT is built on the main assumption of the marginal value theorem,
which states that a forager will only leave a patch once it is satisfied
or once the harvest rate of the patch falls below the average harvest rate of
surrounding patches (Krebs et al. 1974, Charnov 1976). The richness of a
patch, however, is not determined solely by the relative amount of food present.
First, there is an opportunity cost associated with foregoing other fitness-
augmenting activities (i.e. territorial defense, ritual display, nest maintenance)
that limit how much time an animal can spend foraging (Brown
1988). Then, during the time available for foraging, a forager must take into
consideration the predation risk of a particular patch by reducing activity
levels (Lima and Dill 1990) and by being vigilant when and where predators
are most likely to occur (Lima 1988, Kotler et al. 2002). To assess predation
risk, a forager will use sensorial cues to determine predator presence
(Lima and Dill 1990) and microhabitat characteristics to assess the feasibility
of an escape attempt, if one is necessary (Spencer et al. 2014). If a forager
is capable of assessing predation risk in this way, it should be possible
to observe differences in foraging behavior between differing microhabitats
(such as open vs. cover) (Lima and Dill 1990). Preference for more open
sites might result from the increased probability of early predator detection
and/or greater success in escape from predators, depending on prey locomotion
mode (Spencer et al. 2014). Thorson et al. (1998) found that fox
squirrels (Sciurus niger) and thirteen-lined ground squirrels (Spermophilus
tridecemlineatus) consumed fewer sunflower seeds in microhabitats that
were farthest from refuges. Seed removal was also lower in patches where
a fake predator doll was present and in patches with substrate differences
(colored tarpaulins, in this case) that made the squirrels stand out. The
ability to detect predators early, a high probability of escape, and low probability
of detection by predators, in part determines the richness of a patch
(Lima 1993). Thus, it is predicted through OFT that a forager will spend
more time in patches with higher levels of profitability (Royama 1970) and,
if capable of assessing predation risk, will be risk-averse (Caraco 1983).
Risk-aversion by foragers can be seen not only in their responses
to changes in microhabitat (Thorson et al. 1998), but also in
responses to highly stochastic patches. Caraco (1982) found that whitecrowned
sparrows (Zonotrichia leucophrys) presented with a choice between
a constant food reward and a variable reward with the same mean
number of seeds consistently chose the constant food reward. In theory,
if a patch is highly stochastic in resource richness, a risk-averse for-
(Caraco 1982). That said, a forager’s energy budget could determine
a forager’s proneness to risk-aversion. When Caraco (1983) presented
food-deprived sparrows with options of a constant food reward and
a variable food reward, the sparrows chose the variable food reward. A
forager is therefore expected to be risk-prone when it is likely to starve
and its best shot at surviving is visiting the highly variable patches. When
the forager is expecting to meet its energy requirements from the normal
variance patches, it should be risk-averse and prefer these patches
(Caraco 1983, Pyke 1984). Average patch richness (in consideration of
predation risk) and temporal variance in patch richness can indicate the
likelihood that a forager will forage at a given patch and for how long.
Under normal energy conditions, it is expected that an animal
will forage optimally by equalizing its harvest rates in all visited patches
(Valone and Brown 1989). Therefore, we can expect that an animal will
forage from both high resource patches and low resource patches until the
harvest rate drops to a particular level (Charnov 1976, Krebs et al. 1974).
This foraging strategy allows a forager to avoid overusing or underusing
patches (Valone and Brown 1989). Newman (1991) argues, however, that
a forager’s true aim is not to maximize its energy gain rate as much as to
simply stay alive. Newman (1991) developed a model that incorporates
predation hazard and risk aversion, where the simple objective function
of a forager is to avoid death. In this model, prey encountered is treated
as a stochastic event, and, just like Caraco (1983), Newman (1991) argues
that an animal’s decisions should depend heavily on its physiological
state. This model predicts that under high predation pressure, a forager
should spend more time in its refuge and accept a lower physiological
condition, where it is not as well-fed or healthy as it otherwise would be.
This model also predicts that patch-residence times (how long a forager
stays at a given patch) should decrease if foraging efficiency increases,
and the forager should spend more time in refuge, in a better physiological
state (Fig. 2) (Newman 1991). According to the marginal value theorem,
patch-residence time should only be affected by distance from refuge
(longer distance equals longer patch residence time) (Charnov 1976).
While fundamentally different from the marginal value theorem, Newman’s
(1991) model still predicts that foragers will preferentially forage from richer
patches, adding however that patch-residence times should decrease
as foragers become more efficient and when predation risk is higher.
When predation risk is not a factor and a forager does not need
to accept a lower physiological state, patch-residence times should (as was
initially predicted) depend mostly on travel time (Charnov 1976). Mammalian
marine predators such as blue whales (Balaenoptera musculus) that
must dive and return to the surface to forage, thus offer a perfect system for
evaluating this particular prediction (Mori 1998). Using velocity-time-depth
recorders and radio transmitters to track blue whale foraging behavior, Doniol-
Valcroze et al. (2011) found (as predicted by OFT) that with predation
risk absent, patch-residence time increased in compensation for increased
travel times. When alternative activities (such as tending to young) or
physiological constraints on travel time are incorporated into the model, as
should be the case with pinnipeds such as Antarctic fur seals (Arctocephalus
gazella), increasing total foraging time (travel time + patch-residence time)
in response to resource scarcity and offspring demands could result in increased
pup mortality (Staniland et al. 2010). Animals that must forage and
tend to their young thus cannot respond to high predation risk and resource
scarcity in the manner predicted by Newman (1991) or in the same way as
blue whales (B. musculus). Instead of simply accepting a lower physiological
state or blindly increasing foraging time in response to resource scarcity,
it is likely foragers like (lactating) Antarctic fur seals (A. gazella) return
to shore in a manner that optimizes the rate of resource delivery to their
young in sacrifice of length of foraging bouts and physiological condition
(see Staniland et al. 2010). Moreover, dive-time will vary across air-breathing
diver species, limiting how long foraging time can be extended in response
to resource scarcity (Stephens et al. 2008). Terrestrial mammals
such as meerkats (Suricata suricatta) similarly must seek to balance how
much food they provide to offspring and how much they eat themselves,
which is influenced by distance between food and pups, gender of foraging
adults, and pup begging behavior; all of which influence patch-residence
time (Brotherton et al. 2001). Patch-residence time is thus affected by species-
and environment-specific conditions and alternative activities and factors
that need to be incorporated into models predicting foraging behavior.
When presented with an environment with multiple resource
patches, however, the question then follows: when should a forager leave
a patch and search for another? According to Krebs et al. (1974), once
the foraging rate drops to a certain threshold, the forager should depart.
One way to determine this threshold is by measuring Giving-Up Time
(GUT), the interval between when the forager last fed and when it leaves
a patch (Croze 1970). Alternatively, a forager might leave a patch when a
certain amount of time has passed or when a fixed number of items have
been obtained (Krebs et al. 1974). The strategy implemented by the forager
depends on the spatial distribution of prey (Iwasa et al. 1981). If prey
items are distributed in a uniform manner, the fixed-time and fixed-number
strategies yield better results than GUT and vice versa (Iwasa et al.
1981, McNair 1982). Essentially, GUT measures how much time a forager
must spend foraging without finding food before it leaves for another
patch (Croze 1970). While the implementation of GUT depends on the
variance of prey in a patch, GUT itself, contrary to predictions first proposed
by Krebs et al. (1974), will be longer in areas with greater patch
Figure 2. Simplification of Newman’s (1991) model. As foraging efficiency increases, a forager should decrease patch-residence time and increase refuge-residence time, allowing it to have a better final physiological state.
richness (McNair 1982, Pyke 1984). Presumably, GUT will be shorter
in patches with high predation risk but longer in instances when the forager
is at risk of starvation and cannot forage in a risk-averse manner.
Foragers must also take competition into consideration when
deciding on a patch. If two species’ diets overlap, they will reduce the
density of the shared prey items in the patches that they share (MacArthur
and Pianka 1966). Competitors can respond by expanding their diets
(Schoener 1974, Pyke 1984), by reducing their temporal overlap (Pianka
1969, MacArthur and Levins 1964), or by reducing their patch use
(MacArthur and Pianka 1966). OFT predicts that animals with fixed caloric
requirements and contingency feeders should respond to a decrease in
food abundance by increasing activity times (Schoener 1974). Newman’s
(1991) stochastic dynamic model, on the other hand, predicts that these
animals will simply accept a lower final physiological condition. Either way,
over evolutionary time, considerable competition for resources between
species results in three dimensions of specialization: food, place, and time,
which promote coexistence (Pianka 1969). Within these dimensions, species-
specific foraging costs and microhabitat preferences, to a large extent,
determine the local distribution and foraging behavior of species, and thus
also promote coexistence. Abu Baker and Brown (2014) showed that these
species-specific costs and preferences could be estimated through the use
of live-trapping and measuring foraging intensity at different adjacent microhabitats.
In essence, each forager should have a competitive advantage
over other foragers under particular conditions (Pianka 1969, Schoener
1974). Determining the conditions that give a forager species a competitive
edge over another can then be used to predict where each species
should forage in higher numbers and where coexistence between competitors
is favored (MacArthur and Levins 1964, Abu Baker and Brown 2014).
Coexistence of competitors is threatened by generalist foragers.
When a species that specializes on a single resource shares this resource
with a generalist, competition by the two can drive resource abundance
down and thus impose risk of extinction over the specialist species
(MacArthur and Pianka 1969). Given that competition can reduce resource
abundance for some species more than others, which then forces those
species into a lower physiological state (Newman 1991), it follows that
foraging behavior should be expected to change. An animal’s foraging
strategy, in response to competition, may switch from risk-averse to riskprone
(Caraco 1983), or from a fixed-number or fixed-time strategy into a
strategy that more closely follows the patterns predicted by the marginal
value theorem and GUT (Iwasa et al. 1981, McNair 1982). Competition
can also occur when the population of a predator increases in response
to the introduction of an alternative prey species, which then leads to a
reduced population of prey that share a particular predator (Holt 1977).
This should also influence the foraging behavior of the prey species by
reducing the time available for foraging for both prey species when the
predator species is most active. Thus, when examining the foraging behavior
of an animal in a given time and space, it is important to evaluate
factors that may not be immediately obvious, such as a recent increase
in the abundance of a similar forager, which can affect foraging behavior.
After incorporating these factors into the model, the question might then
be, what is the best way to test the assumptions of OFT to learn about
an animal’s foraging behavior? Measuring GUT offers insight into how
long a forager will search for food before “giving up” and searching for
richer patches (Croze 1970), but most studies that focus on OFT choose
to measure food consumption using artificial patches to gain insight into
when a patch’s harvest rate falls below the average rate of the area.
Chapter 2: Giving-Up Density
OFT predicts that an optimal forager will reduce the resource
density of the patches it forages from down to the same quitting harvest
rate (the rate of energy gain when a forager decides to leave a patch),
assuming that its harvest rate is correlated with fitness (MacArthur and
Pianka 1966, Charnov 1976, Valone and Brown 1989). Measuring the density
or amount of resources left in an artificial patch after a foraging bout is
one way of measuring a forager’s quitting harvest rate (Brown et al. 1997)
and testing the prediction of OFT (Brown 1988, et al. 1992, Valone and
Brown 1989). Measuring this remaining food density, or Giving-Up Density
(GUD), can provide information on the costs and benefits a forager experiences
from foraging at a particular patch (Bowers and Breland 1996), how
time of day affects foraging behavior in different microhabitats (Jacob and
Brown 2000), which species are the most efficient competitors in a given
community (Brown et al. 1997), how foraging strategies and food preferences
change in response to the distribution and abundance of food
resources
(Brown and Morgan 1995), how different species respond to variations
in resource density differently (Abu Baker and Brown 2009), and even
how moon phases can influence foraging behavior (Kotler et al. 2002).
Understanding the factors that affect foraging behavior can be particularly
important for species that tend to also disperse seeds as they forage.
By measuring GUD, it is possible to gain information on a forager’s
ability to assess patch richness (Thorson et al. 1998), which will
invariably affect its foraging strategy (Iwasa et al. 1981, McNair 1982). To
determine the foraging strategies used by several desert rodent species
and a few bird species in Tucson, Arizona, Valone and Brown (1989) measured
how these rodents and birds foraged in low variance environments
and high variance environments. The foragers were presented with pairs
of trays that differed in seed density of buried millet, and it was predicted
that one tray’s GUD should be a good predictor of the adjacent tray’s
GUD if the forager was highly capable of assessing patch quality (adjacent
trays treated as separate patches). In this experiment, different species
varied in their abilities to equalize GUDs between the adjacent trays, with
most of them being less capable of doing so in the high variance environments.
Furthermore, by comparing the ratio of resource densities in the
adjacent trays before and after a foraging bout, Valone and Brown (1989)
were able to determine the foraging strategy used. Foragers that followed
a fixed-time strategy, which predicts that they will spend the same amount
of time foraging per patch, could be pinpointed when the ratio of seed
density between the adjacent trays before and after a foraging bout remained
the same. When foragers underutilized rich patches relative to the
poor patches, this suggested that the foragers were learning about their
environment as they foraged, and hence were categorized as Bayesian
foragers. When foragers overutilized rich patches relative to poor patches,
this suggested that the foragers were assessing patch richness by
monitoring their energy-intake rate, and were hence categorized as rate
assessors. Finally, when foragers utilized both patches equally, they were
categorized as prescient optimal foragers (MacArthur and Pianka 1966,
Charnov 1976). How well a forager can assess patch richness and hence
how it forages may also be tightly linked with the sharpness of the foragers’
senses (Valone and Brown 1989). Hence, if an animal is found to
forage in a prescient or Bayesian manner, the forager is likely aided by a
strong sense of smell, which some animals, like squirrels (Sciurus spp.)
(Stapanian and Smith 1984, Jacobs and Liman 1991) and agoutis (Dasyprocta
spp.) (Smythe 1978), also use to retrieve seeds cached earlier.
Evaluating a forager’s ability to assess patch richness is based
on more than being able to assess food abundance and density: patch
richness is, in part, determined by costs (such as predation risk) associated
with foraging in a given patch (Lima and Dill 1990). Foragers will use
their senses to pick up visual and olfactory microhabitat cues to assess
predation risk and in turn decide where to forage (Thorson et al. 1998,
Spencer et al. 2014). A forager’s microhabitat preferences can be influenced
by food availability, predation risk, and climatic conditions (Riginos
2015), which can be determined by measuring GUDs. For example, Bowers
and Breland (1996) measured the GUD of gray squirrels (S. carolinesis)
over an urban-rural gradient to investigate the effect of urbanization
on squirrel populations and their foraging behavior. Mean individual GUD
was found to be lower at sites closer to urban areas, which suggests that
there is less available food for squirrels in urban areas relative to rural
areas. However, squirrel population density was also found to be higher
in urban areas relative to rural areas. Low GUDs and high squirrel density
suggests that the cost of foraging in the resource-poor urban areas
is outweighed by the reduced predation risk in this microhabitat. A forager’s
perception of patch richness is therefore influenced by costs, such
as perceived predation risk, and benefits, such as high resource density.
A forager can assess the predation risk associated with a particular
microhabitat by picking up on sensorial cues that are signs of predator
presence (Lima and Dill 1990) and/or microhabitat characteristics that facilitate
early-predator detection and escape attempt feasibility (Spencer et
al. 2014). Jacob and Brown (2000) measured the GUD of common voles
(Microtus arvalis) to investigate how differences in microhabitat (more specifically,
the effect of reduced cover) and time of day (day vs. night) influence
the rodents’ foraging behavior. Not only did voles have higher GUDs
in mowed grasslands than unmowed grasslands, which supports previous
work on predation risk assessment (Thorson et al. 1998), but time of day
was found to interact strongly with microhabitat, presumably in response to
the effectiveness of owl predators in the mowed grass microhabitat at night
and weasels in the unmowed grass microhabitat during the day (Jacob and
Brown 2000). In this study, measuring GUD provided information on the
cues (microhabitat and time of day) voles (and presumably other animals)
use to assess predation risk; this information could be applied to maintain a
healthy vole population. It also teaches a lesson on the importance of spatial
heterogeneity in habitats for species coexistence, as well as a reminder of
the dimensions of niche specialization (see Pianka 1969, Schoener 1974).
Niche specialization is important for coexistence between foraging
competitors (Pianka 1969, Abu Baker and Brown 2014). Since GUD
is a measure of foraging efficiency, it can be used at a community level to
measure competitive ability between species (Halliday and Morris 2013)
and intraspecific competition (Berger-Tal et al. 2015). Brown et al. (1997),
for instance, measured GUDs for two gerbil species (Gerbillus allenbyi and
G. pyramidum) and the crested lark (Galerida cristata) that compete for the
same food, to find the more efficient forager in four different microhabitats in
the Negev Desert, Israel. In this experiment, obtaining a low GUD demonstrated
a forager’s ability to efficiently harvest seeds at low abundances
in a particular microhabitat, which provides it with an advantage over the
less efficient foragers with higher GUDs. Brown et al. (1997) predicted that
each of the three foragers would have an advantage over the two others in
one way or another. It could be by each having a time and space in which
they reduce food to the lowest GUD, by each species reducing a particular
food type to the lowest GUD, or by compensatory competitive advantage
(e.g. lower travel costs, higher foraging efficiency at high resource patches).
They found that gerbils reduced food density more in the bush and
semi-stabilized sand habitats over open and stabilized sand habitats. With
larks, the opposite pattern was observed. However, despite this clear habitat
preference, gerbils were still able to reduce food density more than larks
in any habitat, during each of the months the experiment took place. While
it is unclear how gerbils and larks coexist in the Negev Desert (authors
suggest diet selection and temporal specialization), the experiment was
successful in showing how differences in habitat affect species differently
(Brown et al. 1997). Said differences can come in the form of predation risk
and in the form of variation in abundance and distribution of food types.
A forager’s diet selection is then a two-step process: first comes
patch selection, and then, within the selected patch, comes food selection
from the available resources (Brown and Morgan 1995). Pulliam (1974)
used theoretical models to predict the optimal switching point (when a forager
should switch from “Food Type A” to “Food Type B” within a patch)
and concluded that acceptance or rejection of a food type depended on the
density of the preferred food type. A year later, Pulliam (1975) concluded
that this might not be the case when there are nutrient constraints; an optimal
forager in these circumstances may exhibit partial preferences instead
of complete acceptance or complete rejection of an alternative food type.
Partial preference refers to the consumption of food types disproportionately
to their occurrence in a given patch (Brown and Morgan
1995). For generalists like the fox squirrel (S. niger), different food types
co-occur within patches but these food types will vary in their encounter
probabilities across patches (Stapanian and Smith 1984). Diet strategies
and food preferences can be teased apart through the use of GUD experiments,
complemented with on-site observation (Emerson and Brown
2012). To investigate optimal switching point and partial preferences,
Brown and Morgan (1995) measured the GUD of fox squirrels in artificial
patches with peanut and/or sunflower seeds at varying food densities.
When the food types co-occurred and high GUDs were found, fox squirrels
were partially selective on sunflower seeds over peanuts, but this preference
was reversed when GUDs were low. Furthermore, when sunflower
seeds and peanuts were presented in separate patches, fox squirrels were
partially selective for the food type with higher initial food abundance, or the
one closest to cover (if they had the same initial food abundance). While
foragers usually have a preferred food type, it is evident that diet selection
is heavily influenced, first by distance to safe refuge, and subsequently by
the abundance of alternative food types. In addition, when the preferred
food type contains secondary compounds, the point at which foragers expand
their diet’s can depend on the amount of the preferred food type they
can safely consume (Molokwu et al. 2011). Food type selection is therefore
a process mediated by species-specific partial preferences on available
food types, in context of their relative abundance and distance from refuge.
Like initial food abundance, other factors such —as substrate
depth and total patch area— influence a forager’s behavior. To investigate
how these factors interact with foraging efficiency, Abu Baker and
Brown (2009) measured the GUDs of mourning doves (Zenaida macroura)
and cottontail rabbits (Sylvilagus floridanus) to compare how these two
species forage when presented with patches that vary in food per unit of
surface area and patches that vary in food per unit volume of substrate.
Given the physiological differences between these two species, varying
substrate depths and substrate areas should reveal how foragers with
different sensorial specialties assess and respond to patch richness.
Abu Baker and Brown (2009) predicted that both foragers should prefer
patches with higher total resource abundance, shallower substrates, and
smaller areas, if they are prescient foragers. In response to variations
in initial food abundance, both mourning doves and cottontail rabbits responded
by harvesting more seeds from richer patches. In response to
deeper substrates, both foragers yielded lower GUDs. However, when
food abundance was also high, both foragers spent more time digging in
the deeper patches than they did in patches with equal depth but lower
food abundance (Abu Baker and Brown 2009). Evidently, substrate
depth is a cost to foraging in a particular patch, much like predation risk
(Bowers and Breland 1996, Jacob and Brown 2000), distance from refuge
(Brown and Morgan 1995, Bowers et al. 1993), and handling time
(Rosenzweig and Sterner 1970). Factors that influence the foraging cost
associated with a patch will invariably have an effect on foraging behavior.
In some systems, even the phases of the moon can affect foraging
behavior. By measuring consumption of seeds overnight and level of
apprehension (time redirected from foraging to predator detection), Kotler
et al. (2002) showed that gerbils spent more time in apprehension behavior
and reduced artificial patches more slowly when there was a full moon relative
to when there was a new moon. With brighter moonlight, gerbils are
more exposed to predators. A slower feeding rate is a tradeoff for foraging
on moonlit nights, which foragers, like gerbils, most likely cannot avoid.
In the eyes of a forager, tradeoffs such as those associated with moonlight
and microhabitat characteristics, influence perceived patch richness.
A forager is faced with tradeoffs associated with each patch
that it must take into consideration each time it forages. GUD is a useful
way to investigate these tradeoffs because, ultimately, it is a cost-benefit
type of experiment. GUD can be used to gain an understanding of a forager’s
ability to assess patch richness and test the assumptions of OFT.
Beyond that, GUD can be used to measure foraging efficiencies under
various natural conditions. Understanding the malleability of an animal’s
foraging behavior when faced with patch heterogeneity is important because
of the impact predators can have on prey distribution and density.
Most of the foragers discussed in these two chapters are not only
seed predators; they are also seed dispersers. Seed predators and dispersal
agents play significant roles in the density and composition of forests
(Janzen 1970, Connell 1971, Howe et al. 1985, Howe and Miriti 2004). Thus,
GUD, by providing us with a cost-benefit analysis for foraging behavior,
also provides us with a tool for investigating seed dispersal
and seed fate.
Chapter 3: Seed Dispersal and Seed Fate
Dispersal is functionally defined as the departure of the diaspore
(seed or seed and fruit) from a plant that may result in the successful germination
and establishment of new individuals (Howe and Smallwood 1982).
The pattern with which diaspores depart from their parent tree is known as
a seed shadow; typically a seed shadow follows a leptokurtic distribution,
peaking in seed density a certain distance from the parent plant (Thomas
et al. 1988, Willson 1993), the degree of which is affected by dispersal
system (Willson 1993, Herrera 1995). Variations in seed shadows may be
caused by multiple dispersal agents (Wenny and Levey 1998) and uplifted
wind-dispersed seeds (Nathan et al. 2002) (Fig 3). Biotic and abiotic
components of an environment (e.g. shade conditions, soil-water potential,
soil nutrient distribution, seed predators) will determine a seed’s likelihood
of germination and subsequent probability of establishment (Schupp and
Fuentes 1995, John et al. 2007). Dispersal of the diaspore can occur in
a variety of ways, including but not limited to: wind-dispersal (Nathan et
al. 2002), animal-mediated dispersal (Wheelwright et al. 1984, Thomas et
al. 1988), explosive dehiscence (Hayashi et al. 2009), and more (Traveset
et al. 2014). Dispersal mechanisms allow seeds to reach territories
that might be more favorable for germination, establishment, and growth.
However, if an adult fruiting plant is evidence that the soil beneath
it is suited for the propagation of its own species, why would a
species invest in mechanisms that drive its seeds away from their origin?
Janzen (1970), while seeking to answer an even more perplexing question
(how do you pack so many [species] into a [tropical] forest?), proposed,
along with Connell (1971), a model that explains how the high level of
plant diversity in tropical forests is maintained; this model explains why
mechanisms of dispersal are indispensable and highly variable, particularly
in tropical regions. The Janzen-Connell Hypothesis (also dubbed the “Escape
Hypothesis” by Howe and Smallwood (1982)) predicts that seeds and
Figure 3. Typical seed shadows follow a leptokurtic distribution with one
peak of seed density found a given distance from the parent tree (A). With
multiple dispersal agents or when wind-dispersed seed are uplifted over
the canopy however, seed shadow distributions can be multimodal (B).
seedlings are afflicted by pest pressure in a negative density-dependent
and distance-dependent manner that reduces their survival rate enough
to prevent tropical environments from being dominated by only a few species
(Fig. 4) (Janzen 1970, Connell 1971). Density-dependent mortality of
seeds and seedlings is predicted to occur because an area of high conspecific
density for a specialist pest is a rich patch, and thus specialist pests
should maximize the amount of time they spend foraging there (Royama
1970). Similarly, areas of high conspecific density are ideal for the growth
of fungi and parasitic oomycetes that specifically target certain species
(Packer and Clay 2000, Bell et al. 2006, Mangan et al. 2010). Since the
hypothesis was proposed over 40 years ago, many experiments have followed
and tested its predictions, with some finding support for the hypothesis
(Clark and Clark 1981, Howe et al. 1985, Packer and Clay 2000, Bell
et al. 2006, Mangan et al. 2010, Swamy and Terborgh 2010) and some
finding evidence and logical conclusions against it (Wright 1983, Hubbell
1979, 1980). To finally lay the debate to rest, reviews and critiques such
as those written by Clark and Clark (1984) and Carson et al. (2008) have
used a tally system to show that there is more evidence for than against
the Janzen-Connell Hypothesis. More recently, Comita et al. (2014) used
a meta-analysis to evaluate the weight of the evidence in favor of the model
from all available studies and found significant support in favor of the
Janzen-Connell Hypothesis. While evidence supporting the notion that negative
density-dependence is strongest in the tropics (Janzen 1970) is lacking
(Comita et al. 2014), ultimately, evidence suggests that there is a selective
force that favors seeds dispersed further away from the parent plant.
Figure 4. Seed density (D) decreases sharply past the cover of the parent tree and
steadily decreases past the crown. The probability of seed survival (P) increases with
a decrease in seed density as a result of density-dependent predators.
nell Hypothesis, the next step is to ask when the effect of negative density-
dependence is of relevant importance (Howe and Miriti 2004). It is not
enough to determine that seeds are most likely to escape predation if they
are dispersed in low conspecific density away from parent trees. Germination
success not only varies across patches spatially and temporally, but the
best site for germination is not necessarily the best site for seedling establishment
and growth (Schupp and Fuentes 1995). In other words, negative
density-dependence is one problem; others are how these seeds are being
dispersed, in what type of patches, and how many per patch type make it
to adulthood (Howe 1989). Janzen’s (1970) claim that dispersal agents are
determinants of the density and spatial distribution of plant species in forests
assumes that dispersal is the most critical determinant of plant demography,
but we also know that plant recruitment rates are strongly influenced
by the biotic and abiotic environment, as well as a seed’s intrinsic traits and
defense mechanisms (Schupp and Fuentes 1995, Dalling et al. 2011). An
evaluation of the factors that play a part in plant demography is thus indispensable
to understanding the full impact dispersal agents have on the
spatial distribution and density of forest species and natural succession.
It is first important to discuss the differences between dispersal
syndromes found in tropical environments to evaluate when seed dispersal
by a particular agent is beneficial to a plant species. Most fleshy fruits in
tropical forests are dispersed either by birds (Wheelwright et al. 1984), bats
(Thomas et al. 1988, Wunderle 1997), arboreal primates (Janson 1983), or
terrestrial mammals (Leigh 1999). Within animal-mediated dispersal, there
is a stark dichotomy between seeds dispersed in clumps and scattered
seeds. Large animals are most often those that disperse seeds in clumps;
these animals will consume various seeds as they forage and defecate
them in a single location (Noble 1975). As Howe (1989) explains, since
clump-dispersed seeds frequently germinate centimeters from each other,
it is likely that they possess more secondary chemicals than scattered-dispersed
seeds. Enhanced defense by secondary compounds would allow
them to stave off more pests, theoretically making dispersal more important
than seed conspecific density in clump-dispersed seeds. However, unless
secondary seed dispersal occurs, saplings in a single clump will compete
intensely with each other, until only one sapling remains and matures to
adulthood (Howe 1980). Secondary seed dispersal might therefore provide
a way for clump-dispersed seeds to escape intraspecific competition.
Scatter-dispersed seeds follow a different fate. Virola surinamensis
(Myristicaceae) is an example of a tropical canopy tree species
that depends heavily on dispersal by birds for seed survival. By placing
V. surinamensis seeds at increasing distances from fruiting adults, Howe
et al. (1985) were able to show that seed survival is up to 44 times more
likely when seeds are dispersed 45 m away from a fruiting adult. Without
seed dispersal, V. surinamensis tree recruitment would be reduced
dramatically, since the seeds and seedlings lack the defenses needed to
deter oviposition by the curculionid weevils (Conotrachelus) responsible
for most seed and seedling mortality under and near fruiting Virola adults
(Howe et al. 1985). Seeds that are bat dispersed can either be scattered,
if they are defecated mid-flight as bats tend to do, or they can be dispersed
in clumps once a bat reaches a roost or perch (Thomas et al.
1988, Gorchov et al. 1993, Wunderle 1997). It is likely that when faced
with higher levels of predation, bats will spend more times at their perches
(Howe 1979), which would result in more clump-dispersed than scattered
seeds. The bat-dispersal system thus offers an ideal system for investigating
the differences between the clump and scatter dispersal forms.
The benefit a species receives from a particular dispersal mode
depends on its seeds’ defense syndrome and how they respond to the
biotic and abiotic components of the environment into which they are dispersed.
Seeds that persist in the soil in an ungerminated state can either
be dormant (physical or physiological barriers deter germination) or quiescent
(seeds will germinate only under favorable conditions) (Dalling et
al. 2011). Species from the genus Cecropia (Urticaceae), for example,
produce notorious pioneer seeds that tend to germinate soon after dispersal
into gaps, but can also survive up to two years in the soil seed
bank (Gallery et al. 2007). The persistence of quiescent seeds, that lack
physical and physiological defenses, is indeed impressive and it is likely
they owe this characteristic to endophyte symbionts (Gallery et al. 2007,
Dalling et al. 2011). How Cecropia species are distributed naturally is likely
a result of interplay between the seed shadow provided by different bat
species, the proportion and survival rate of scattered vs. clump dispersed
seeds, and, ultimately, the number of seeds that land in patches ideal for
germination. In addition, seed mortality by fungal infection will vary by
fungi suite and seed species (Gallery et al. 2010). Thus, while knowing
the dispersal syndromes a species utilizes provides insight into where its
seeds can be found, ultimately, factors such as the soil nutrients (John
et al. 2007), soil-borne fungal pathogens (Gallery et al. 2010), and canopy
gaps and clearings distributions (Raich and Khoon 1990, Dalling et al.
2002) will be the factors that can more accurately predict where seedlings
are likely to establish and mature. While negative density-dependence influences
the density and spatial distribution of plants (Comita et al. 2014),
it is clear that this effect does not solely determine plant demography.
In conclusion, estimating seed shadows is not enough; studies
that explore post-dispersal fate and link seed dispersal with seedling establishment
are necessary for projections of natural restoration to be accurate
(see Schupp and Fuentes 1995). Exemplary studies such as those by Howe
et al. (1985), Forget (1990), Herrera et al. (1994), and Wenny (2000) have
addressed this disconnect between seed dispersal and seedling establishment
in V. surinamensis, Vouacapoua americana (Fabaceae), Phillyrea
latifolia (Oleaceae), and Ocotea endresiana (Lauraceae), respectively, and
provide true insights into the relative importance of seed dispersers, seed
and seedling predators, and seed shadows. By linking seed dispersal with
seedling establishment through a post-dispersal study, information on possible
secondary seed dispersal and the conditions that yield highest seedling
establishment rates and the distribution of these seedlings can be obtained.
For example, while the ecological relationship between squirrels
(Sciurus) and oaks (Quercus) is well understood, burial of acorns by squirrels
does not necessarily equate higher germination rates (Fox 1982). By
following the fate of oak acorns post-burial, Barnett (1977) found lower
germination rates for acorns buried by squirrels as a result of notched embryos.
It would seem that squirrels arrest germination in buried acorns to
prevent them from transferring their nutrients into a taproot. Post-dispersal
seed fate can be investigated by use of a thread marking method and/
or camera traps to keep track of the fate of individual seeds. By using
both thread-marking and camera traps, Forget (1990) was able show how
agoutis (D. leporina) and acouchies (Myoprocta exilis) function as dispersal
agents for V. americana seeds, scatterhoarding over 70% of them in
the immediate vicinity, which allowed some seeds to escape high predation
rates on the surface. The survival of buried seeds, on the other
hand, can depend on food availability on the surface. Post-dispersal predation
by the very same caviomorph rodents that bury these seeds tends
to decrease mid-fruiting season, thus promoting seedling establishment
(Smythe 1978, Forget 1993). Mast fruiting may be an adaptation that allows
rodent-dispersed seeds to escape total predation (Jansen and Forget
2001). Once the fruiting season has passed, however, agoutis and
acouchies return to eat the cotyledons and seedlings of the seeds they
once buried (Forget and Milleron 1991, Forget 1992). Not all seeds and
seedlings have equal value, however; large seeds tend to be cached in
lower densities than small seeds (Stapanian and Smith 1984). Since optimal
seed predators should preferentially forage from sites with higher
resource density (MacArthur and Pianka 1966, Royama 1970), caching
seeds in low densities is a way to safeguard the possibility of losing all
cached goods in a single foraging bout. This response should be exacerbated
in times of resource scarcity, when all seeds increase in value
(Jansen and Forget 2001). It should also be noted, however, that the nutritional
content of seeds tends to decrease with time (Post 1992), which
means that patch richness should also decrease over time when no seeds
are removed or replenished. Since post-dispersal predation and caching
density are both affected by food availability, the season in which these
studies are conducted, as well as the regions in which they take place,
will invariably affect the rate of seedling establishment and the density.
Investigating post-dispersal seed fate should also go past termination
of germination potential because infested seeds might still serve to
satiate seed dispersal agents. For example, gray squirrels (S. carolinensis),
upon distinguishing sound acorns from infested acorns, preferably eat
those with weevil larvae inside and cache only the sound ones (Steele et al.
1996). Other rodents, like the white-footed mouse (Peromyscus leucopus),
prefer sound acorns, but are unable to distinguish them from infested ones
(Semel and Andersen 1988). Then there are birds like Clark’s nutcracker
(Nucifraga columbiana) and the blue jay (Cyanocitta cristata) that are able to
differentiate between sound, edible seeds and infested, inedible ones, and
preferentially consume those that are sound (Vander Wall and Balda 1977,
Dixon et al. 1997). While not a new idea, this highlights the fact that the way
in which different animals affect seed dispersal and seed fate is also bound
to vary between dispersal agents. In the case of oaks, not all terrestrial
mammals will exclusively eat sound acorns (Semel and Andersen 1988,
Steele et al. 1996), which means that one species might exert a stronger influence
on acorn dispersal than another. All this information can be used to
focus conservation efforts on those species indispensable for forest growth.
As is developed further in the next chapter, restoration efforts in
Monteverde, Costa Rica aim to reconnect forest fragments by planting native
plant species (Lauraceae species in particular) that are indispensable
to the survival of native animal species. If it is of interest to ensure high Lauraceae
seedling establishment rates, should conservation efforts focus on
the black guan (Chamapetes unicolor), a clump-disperser, or resplendent
quetzals (Pharomachrus mocinno) and three-wattled bellbirds (Procnias
tricarunculata), which are all scatter-dispersers (Wenny and Levey 1998)?
Perhaps wire frames should be used to protect newly established seedlings
from predation or perhaps the number of terrestrial seed predators should
be reduced using animal traps. Making the right decision will depend on
our ability to determine a plant species’ most vulnerable stage. This information
will come from investigating the impact each dispersal agent has
on dispersal, where and how these seeds are being dispersed, the possibility
of secondary seed dispersal, and the intensity of negative-density
dependence on seed and seedling mortality across time and space. It
is a grave mistake to assume that the pattern of primary seed dispersal
is equivalent to the pattern in which seedlings are naturally established.
Chapter 4: Introduction to Costa Rica, Lauraceae, and Study
With one of the highest levels of biodiversity density in the world
(~500,000 species in 51,000 km2) (MINAE and SINAC, 2003), Costa Rica
is a Neotropical country recognized for spearheading conservation efforts
and sustainability in Central America (Nadkarni and Wheelwright 2000,
Vargas 2007, Calvo-Alvarado et al. 2009,). Costa Rica has not always
been known for its progressive environmental policy, however. Up until the
1980s, demand from the United States and Europe for massive amounts of
beef and cash crops (particularly timber, coffee, cotton, sugar, and bananas)
led the local farmers to ravage the rich primary forests in search of arable
land (Myers and Tucker 1987). Demand for Costa Rican beef plummeted in
the 1980s (Calvo-Alvarado et al. 2009), in part due to boycotts by environmentalists
(Miller 2012), but decades of relentless deforestation left Costa
Rica with fragmented forests (Sánchez-Azofeifa et al. 2001) invasive exotic
grass species in abandoned pastures (Nadkarni and Wheelwright 2000,
Lisa 2012,), and approximately 3,500 animal and plant species in danger
of extinction (Klugman 2011). In response to pressure from environmentalists,
the Costa Rican government introduced subsidized reforestation and
forest management practices, a permit system (under the Forest Law of
1996) to restrict timber extraction, and a Payments for Environmental Services
Program, which provided incentive for private conservation actions
(Calvo-Alvarado et al. 2009). All these movements successfully decreased
the deforestation rate in Costa Rica, but it soon became clear that beloved
flagship species like the three-wattled bellbird and the resplendent quetzal
would remain in danger of extinction as long as any one of the ecosystems
that form their migratory routes remained fragmented (Powell and Bjork
1995, 2004, Nadkarni and Wheelwright 2000, Rojas and Chavarría 2005).
To improve habitat connectivity for species threatened by forest
fragmentation, the Bellbird Biological Corridor (BBC) initiative was formed
in 1992 (Fig. 5) to reconnect the mangroves on the Gulf of Nicoya (sea level)
with the cloud forests of Monteverde (~1850m) (Ryan 2012). Monteverde
is of particular interest to the BBC because of the high number of species
that inhabit the zone (Nadkarni and Wheelwright 2000). In addition to restoration
efforts, the BBC initiative monitors three-wattled bellbird populations
and carries out reforestation projects focused on Lauraceae species in the
Monteverde region (FCC, no date). At least 18 threatened and endangered
bird species, including the bellbird, feed on these lipid-rich fruits (Wheelwright
et al. 1984). Out of these 18, four species, the three-wattled bellbird,
resplendent quetzal, emerald toucanet (Aulacorhynchus prasinus), and
mountain robin (Turdus plebejus) are recognized as primary dispersers
of the Lauraceae seed, via regurgitation. A fifth, the black guan, disperses
the seeds by defecation (Wheelwright 1991). Thus, not only do these
birds depend on the fruit for survival, these Lauraceous trees depend on
the birds for seed dispersal (Nadkarni and Wheelwright 2000). While the
seeds themselves can achieve equal germination rates irrelevant of dispersal
agent (included here, manual removal from endocarp by experimenters),
germination is not achieved if the pericarp is still attached (Wenny
2000). One of these dispersers, the three-wattled bellbird however, may be
more critical to Lauraceae seedling recruitment than others (Wenny 2000).
Figure 5. Map of the Bellbird Biological Corridor (orange) and surrounding private
reserves (Chinchilla 2015).
A dispersal agent that provides higher seedling establishment
rates for O. endresiana and other Lauraceae species would be particularly
important for Lauraceae restoration efforts. Wenny and Levey (1998)
found that four out of the five dispersers regurgitate/defecate most of the
seeds consumed within 20m from their source. Male three-wattled bellbirds,
on the other hand, dispersed most seeds (59%) to sites over 40m
away from their source. Most of these seeds landed in gaps (52%), where
seedling recruitment was higher. Together, these five dispersers give O.
endresiana and other Lauraceous tree species in Monteverde a bimodal
seed shadow. Later, Wenny (2000) expanded on this study by investigating
the post-dispersal fate of seeds and found that at least 50% of
seed removal could be attributed to small rodents. Seed removal rates
were not significantly higher in gaps than understory. In addition, his experimentally
buried O. endresiana seeds failed to germinate and he found
no evidence for scatterhoarding or secondary seed dispersal. In 2005,
Wenny (2005) would expand on this study again and find no evidence for
secondary seed dispersal in four more Lauraceous species (O. meziana,
Pleurothyrium palmanum, Beilschmiedia costaricensis, and a Persea sp.).
While seed burial may not be advantageous to O. endresiana, the possibility
of squirrels or caviomorph rodents caching Lauraceae seeds is not
excluded. The existence of a seed dispersal system does not require, nor
is it evidence for, a mutual evolutionary benefit to both participants (Herrera
1995). In the montane cloud forest where Wenny (2000, 2005) carried out
his studies, agoutis are much less common than they are further down
in Monteverde. As has been aforementioned, agoutis are famous scatterhoarders
(Smythe 1978) that often return to burial sites to consume their
buried seeds (Forget 1992, 1997), or, if the seeds have germinated, their
expanded cotyledons (Forget and Milleron 1991). It is possible that at lower
altitudes, in the premontane wet forests of Monteverde, agoutis play a
more active role in the seed removal and dispersal of Lauraceous species.
Investigating the relationship agoutis (Dasyprocta punctata) and
other terrestrial foragers have with Lauraceae species is of critical importance
because of the central role the Lauraceae family has in restoration
efforts in Monteverde (Nadkarni and Wheelwright 2000). Given the history
of deforestation of Costa Rica (Sánchez-Azofeifa et al. 2001), connecting
forest fragments entails reforesting abandoned pastures and agricultural
lands with native species. Without human intervention, wind-dispersed
species, blown downwind from far off places, will be the first to colonize
these sites (Howe et al. 2010). Colonization by tall, wind-dispersed species
thus sets the stage for natural succession by providing perches and
cover for seed-dispersing animals (Janzen 1988). The establishment of
persistent, colonizer species, such as Juniperus virginiana, in more temperate
regions, provides cover for successional species, such as oaks,
which will attract seed dispersers with their cover and food (Yarranton
and Morrison 1974). Natural succession takes centuries, however, and a
more efficient way to restore these abandoned sites in the tropics is by
connecting forest fragments, through the creation of regeneration nuclei,
by planting tree “islands” (Cole et al. 2010, Holl et al. 2011). By excluding
cattle from these islands, seedling survival from incoming animal-dispersed,
late-successional species is enhanced (De la Peña-Domene et
al. 2013), particularly if these tree islands are composed of animal-dispersed
species (De la Peña-Domene et al. 2014), thus bypassing the
lengthiness of natural succession (Cole et al. 2010). Given the various
agents that disperse Lauraceae seeds (Wheelwright 1991, Wenny 2000),
planting them not only protects the species involved in this dispersal mutualism,
but also attracts other animals seeking cover and respite from
open pasture, along with the seeds they may carry (see De la Peña-Domene
et al. 2013). If agoutis (D. punctata) also interact with the removal
and/or dispersal of Lauraceae seeds, their effect must be evaluated, as
it could be helpful or detrimental to Lauraceae seedling establishment.
The objective of my study was to expand on Wenny’s work
and further explore the possibility of secondary seed dispersal for Lauraceous
species in Monteverde. The species studied was O. floribunda
(Lauraceae), a canopy tree species known for its highly aromatic leaves
and bark. Using a GUD experiment, a seed tagging experiment, and a
camera trap, I sought to answer the following questions: (1) do terrestrial
foragers remove regurgitated seeds and/or immature fruits from the forest
floor? (2) Is seed removal more intense in forest gaps? (3) Is seed removal
more intense during the day? (4) Are regurgitated seeds preferred
over seeds with manually removed pericarp 5) Do regurgitated seeds
experience the same rate of infestation as immature fruits found on the
ground? (6) Are regurgitated seeds cached? Since agoutis (D. punctata)
are common in the study site, I predicted that seed and fruit removal
would occur during the day (since agoutis are diurnal (Smythe 1978))
and under forest canopy cover. In addition, I predicted that seed removal
would be strongest for regurgitated seeds. While I could not confirm
this, I found it reasonable to predict that regurgitated seeds carry a specific
scent that foragers could detect. I also predicted that infestation rates
would be highest for regurgitated seeds because they were collected without
their pericarps and had thus been exposed without this layer to pests
for longer. Lastly, with the seed tagging experiment, while Wenny (2000,
2005) did not find evidence for scatterhoarding from his experiments in
the montane cloud forest, I predicted that a small fraction of regurgitated
seeds would indeed be cached in the wet premontane forest. While the
results of these experiments are preliminary in nature, they nonetheless
add to our understanding of the post-dispersal fate of Lauraceae seeds,
which is of critical importance for restoration efforts led by the BBC.
Chapter 5:Materials and Methods
STUDY SITE—This study was conducted within the Monteverde
Ecological Sanctuary (MES), located at about 1300 meters above sea lev-
Figure 6. Model of the GUD experiment. One plot was established under canopy
cover and one in the open.
els (84°49′′ W, 10°18′′ N) during July and August 2014. The MES is a private
reserve and member of the Costa Rican Conservation Foundation (CRCF),
which, as part of the BBC initiative, aims to reconnect forest patches on
the Costa Rican Pacific slope. The objective of the CRCF is to reforest and
protect areas deemed indispensable for the survival of the three-wattled
bellbird, while educating the public about the importance of this mission. As
part of the CRCF, the MES collaborates in reforestation projects and uses
various trails and a sustainable banana plantation found within the reserve
for environmental education. The MES is located in premontane wet forest
on the Pacific slope of the Tilarán Mountain range in Cerro Plano, Monteverde,
Costa Rica. The environment is characterized by dense, tropical
evergreen vegetation with a low closed canopy, high levels of precipitation
(2000 – 2500 mm of annual precipitation), large biomass of epiphytes, and
dominance of a few plant families, including the Lauraceae (Holdrige 1967,
Golley et al. 1969, Nadkarni and Wheelwright 2000). In addition, while premontane
wet forests in Costa Rica have been heavily deforested (75%),
are highly fragmented, and together cover only about 7.5km2 of Costa Rica’s
land, they form part of the migratory cycle that three-wattled bellbirds
and resplendent quetzals follow every year (Powell and Bjork 1995, 2004).
GIVING-UP DENSITY EXPERIMENT—Two 10 m x 10 m x 10 m
triangular plots were established within the MES, one under canopy cover
and another in an open area (Fig. 6). It was important that the open
plot be adjacent to forest cover and within the private reserve to ensure
that both plots were equally likely to be encountered by the same animals.
I established this plot at the edge of one of the banana plantations
where I found a vacant plot adjacent to forest cover. For the canopy plot,
I traveled 100 m away from the open plot into the forest and established
it at the edge of an open area created by a trail. The seeds and fruits
of O. floribunda were collected from the forest floor underneath several
O. floribunda adults within the private reserve. Fruits were collected when
they were bright green and without visible damage. Regurgitated seeds
were collected when I was not able to crush them between my fingers
(a test performed to eliminate heavily infested or rotten seeds from the
sample) and when there was no visible damage. Once collected, some
of the fruits were taken back to a nearby private residence where a
pocketknife was used to remove the pericarp, to create a control treatment
composed of seeds that were manually removed from their fruit.
The seed treatments (20 fruits, 20 regurgitated seeds, and 20
control seeds) were placed at the three nodes of each plot on the following
dawn. Plots were checked every day at dawn and dusk for consumption,
and fruits and seeds were replenished to a total of 20, if consumption had
occurred. To eliminate the effect a particular node might have on GUDs,
the treatments were swapped clockwise across the nodes once per day
following data collection at dawn. In addition, a Bushnell® camera trap
was placed within the plots, aimed at one of the nodes, to photograph
foraging activity. The camera settings were set to use no flash and makno
sound while taking photographs. Initially, the location of the camera was
swapped every day at dawn between the plots and randomly across the
nodes. By the second half of the experiment, however, it became apparent
that the regurgitated seeds at the open sites attracted more foragers than
the other treatments. Thus the camera trap was then left aiming at this
treatment. To keep the food samples as fresh as possible, fruits and seeds
were replaced with newly collected fruits and seeds every 3-4 days. At the
end of the experiment, all seeds and fruits were collected, sliced open with
a pocketknife and examined for the presence of parasites. The state of
each seed was classified with a simple dichotomy: infested or uninfested.
FATE OF SEEDS EXPERIMENT—Following Wenny’s (2000)
procedure, 50 cm of unwaxed dental floss was glued to the seed coat of
40 O. floribunda regurgitated seeds using Superbonder Instant Adhesive®,
and 50 cm of pink flagging tape was tied to the distal end of the dental
floss. The following day, at dawn, from a specific point within the forest,
with 10 seeds per cardinal direction, the seeds were placed 3-5 m away
from each other in a straight-line (Fig. 7). This setup was used instead
of random scattering in order to minimize the chance of losing seeds in
the expanse of forest. Seeds were checked for consumption/caching every
other day at dawn. A handheld Garmin GPSMAP 60CSx was used to
mark the location of all the seeds. At the end of the experiment, all seeds
were collected, sliced open with a pocketknife, and examined for the presence
of parasites. The state of each seed was classified into one of five
categories: “untouched and uninfested,” “untouched and infested,” “buried
and uninfested,” “buried and infested,” and “consumed.” Seeds used
for this experiment were also collected from within the private reserve.
STATISTICAL ANALYSIS—Welch’s t-test was used to test mean
GUD differences in seed removal between seed treatments and microhabitats.
The X2 test of independence was used to evaluate the extent to
which the observed proportions of infested to uninfested seeds in the GUD
experiment deviated from expected values. Two-sample z-tests of proportion
were used to compare infestation rates between seed treatments. The
software program RStudio version 0.96.122 (R Development Core Team,
2012) was used to carry out all statistical analyses and create graphs.
Figure 7. Model of seed tagging experiment. Seeds were scattered in four directions
from one set point under canopy cover with a minimum 3 m distance between each
of them.
Results
CAMERA TRAP—A camera trap was used throughout the GUD
experiment to record the species of mammals and birds visiting the experimental
site. None of the animals photographed by the trap were the
aforementioned primary dispersers. The camera trap captured seed removal
once, and the forager was a Central American agouti (D. punctata)
(Plate 1). This species was also the most common animal captured by the
camera trap (6/26 sightings) (Table 1). Ring-tailed coatis (Nasua nasua),
the second most common visitors (5/26 sightings), had been observed
seemingly interested in O. floribunda seeds and fruits, and one of them
was even photographed smelling regurgitated seeds, but consumption by
ring-tailed coatis was not observed or recorded. The same day the agouti
was photographed consuming regurgitated seeds was the day with highest
seed removal (13 regurgitated seeds removed). Central American agoutis
(D. punctata) have long been recognized as granivores (Smythe 1978),
but consumption of O. floribunda seeds by this species had not been reported
in the scientific literature. The same agouti, seconds after consuming
some regurgitated seeds, attempted to bury a seed in the immediate
vicinity (Plate 2). When the site was visited that afternoon, a very shallow
hole (~ 1 cm deep) was found and bits of chewed up seed were present
immediately adjacent. While an unsuccessful burial attempt, this behavior
suggests agoutis engage in secondary seed dispersal for this species.
Table 1. Species captured by camera trap.
Plate 1. Dasyprocta punctata consuming an O. floribunda regurgitated seed.
Plate 2. Dasyprocta punctata in an attempt to bury regurgitated O. floribunda seed
fragments.
Daytime seed removal was recorded multiple times, though no significant
difference between mean GUD was observed between microhabitats
for regurgitated seeds (t = 1.13, df = 12.13, p-value = 0.28) or control
seeds (equal means). Within the canopy microhabitat, the difference in
mean GUD between regurgitated seeds (19.5) and control seeds (19.83)
was not significant (t = -1.17, df = 14.94, p-value = 0.26) (Fig. 8). Within
the open microhabitat, the difference in mean GUD between regurgitated
seeds (18.17) and control seeds (19.83) was also not significant (t
= -1.44, df = 11.46, p-value = 0.18) (Fig. 9). These results suggest that
the only condition necessary for seed removal of O. floribunda seeds on
the ground is for the pericarp to be have been removed, the means of
how is not of particular importance to foragers. Given that agoutis (D.
punctata) were the most commonly seen mammal in the area (personal
observation), the most commonly photographed animal, and a diurnal
forager (Smythe 1978), it is permissible to conclude that agoutis were
responsible for most, if not all seed removal in this experiment. However,
it is worth noting that because consumption events were rare and
non-consumption events were included in the analysis, the mean GUDs
were all very close to the initial food density (20 items), with regurgitated
seeds in the open microhabitat during the day having the lowest mean
GUD (18.17). It is therefore possible that the true differences in seed removal
between seed treatments and microhabitat could have been unmasked
if the study had been extended to include a larger sample size.
Figure 8. Mean GUD under canopy cover during the day. Error bars represent standard
error. Difference between the means (Regurgitated = 19.50, Control = 19.83) is
not significant (t = -1.17, df = 14.94, p-value = 0.26)
Figure 9. Mean GUD in the open during the day. Error bars represent standard error.
Difference between the means (Regurgitated = 18.16, Control = 19.83) is not significant
(t = -1.44, df = 11.46, p-value = 0.18).
At the end of the GUD experiment, the remaining seeds and fruits (40
from each group) were dissected to assess infestation rates (Fig. 10). Regurgitated
seeds had the highest incidence of infestation by arthropods
(72.5%), followed by control seeds (57.5%) and seeds in intact fruits
(40%). The observed proportions of infestation deviate from predicted values
(X2 = 8.62, df = 2, p-value = 0.013). In addition, regurgitated seeds
had a higher infestation rate than fruits (z = 2.9299, p-value = 0.003).
Figure 10. Final seed state. The difference in proportion of infested seeds to uninfested
seeds between treatments is significant (X2 = 8.62, df = 2, p-value = 0.013). Regurgitated
seeds have a higher infestation rate than fruits (z = 2.93, p-value = 0.003).
SEED TAGGING EXPERIMENT—Out of the 40 seeds tagged
and left under canopy cover, after ten days (duration of experiment), six
were buried (three of which were infested with parasites) and one was
consumed. Out of the six buried seeds, four were buried under considerable
leaf litter, in a shallow opening in the soil, while two were buried
(~2 cm) under compact soil. Most seeds were not touched throughout
the experiment (33/40) (Fig. 11). The fact that the proportion of buried uninfested
seeds to buried infested seeds is equal (3:3) suggests that the
animals responsible for caching these seeds are unable to determine
infestation rates or are unable to detect infestation by a particular pest.
Like the GUD experiment, the seed tag experiment’s regurgitated
seeds had a high rate of infestation (68%), which once again suggests
that most seed viability of O. floribunda is terminated not by
granivore vertebrates, but through infestation by arthropods. It is not
possible to determine whether the consumed seed was infested or
not at the time it was consumed. Infested seeds either contained developing
larvae of hymenopterans or other unknown species (Plate
3). Like the GUD experiment, the seed tagging experiment was limited
by its short duration and the relatively low number of seeds used.
Plate 3. Wasp emerging from an infested regurgitated O. floribunda seed.
Discussion
My findings suggest that in the wet premontane forests of Costa
Rica, O. floribunda seeds are subject to secondary seed dispersal (Fig. 11).
The dispersal agents responsible here are most likely Central American
agoutis (D. punctata), as suggested by camera trap photographs (Plate 1
and 2) and previous literature (see Smythe 1978), though discarding the involvement
of other dispersal agents would be premature. This finding contrasts
with Wenny’s (2000, 2005) findings, where he did not find evidence
for secondary seed dispersal. Certainly, differences in the population size
of agoutis (D. punctata) between my site and Wenny’s (2000) could explain
differences in the results of our seed tagging experiments, but while our
tree species are closely related, and found in the same environments, differences
in our experiments could be a consequence not of experimental
site, but simply of seed species used. Be that as it may, the impact secondary
seed dispersal has on O. floribunda seedling establishment remains
to be evaluated. In light of Wenny’s (2000) findings on the effect of experimental
burial on O. endresiana germination, it is likely that secondary
dispersal of O. floribunda seeds yields no benefit to these seeds. If this is
the case, and agoutis respond to high resource density, by caching, as they
do with Carapa procera seeds in French Guyana (Forget 1996), agoutis
may represent a particularly strong deterrent of Lauraceae seedling establishment,
given that, unlike other seed predators, agoutis will continue
to remove seeds —without any added dispersal benefit— post-satiation.
Figure 11.The final fate of regurgitated seeds from the seed tagging experiment.
Herrera (1995) argues that plant-dispersal systems do not require,
nor are they evidence for, a mutual evolutionary benefit for all participants.
In systems like the Lauraceae, where there are multiple dispersal
agents (Wenny 2000), it is important to evaluate the effect and relative
importance of seed dispersal by each of the agents involved. A species’
seed shadow pattern is not equivalent to its pattern of seedling establishment
(Schupp and Fuentes 1995), and seed dispersal does not always
result in higher germination rates (Barnett 1977, Fox 1982). Burial may
allow seeds to escape surface predators (Forget 1990), but such seed
escape is pointless if it does not result in germination. The seed tagging
experiment should be carried out again with more seeds (Forget (1990)
used 185 and Wenny (2000) used 923) to determine mean burial depth.
On-site camera traps should also be used to verify the identity of species
that bury O. floribunda seeds. Mean burial depth can then be used to carry
out a germination assay experiment to confirm that, like O. endresiana,
burial prevents germination (Wenny 2000). If it can be demonstrated that
agoutis bury O. floribunda seeds without any added dispersal benefit, then
perhaps agouti populations should be monitored in areas undergoing restoration
focused on the Lauraceae, in Monteverde, to mitigate the loss of
Lauraceae seeds to this seed predator. It should be noted however that
the exclusion of any native species from a restoration site comes with
significant ecological consequences. Agoutis should only be excluded or
controlled when it is indisputably clear that doing so will only benefit the
restoration movement (not just Lauraceae species), without inhibiting the
dispersal of other plant species or compromising the populations of other
animals (such as jaguars and ocelots) that depend on agoutis for food.
Microhabitat had no influence on O. floribunda seed removal.
This suggests that the differences in predation risk between microhabitats
are not considerable enough for differences in foraging behavior to be detected
through GUDs. According to OFT, foragers like agoutis, which have
considerable sensorial capabilities (Smythe 1978), should be able to efficiently
assess patch richness (Valone and Brown 1989) and reduce equal
patches to the same quitting harvest rate, or GUD (MacArthur and Pianka
1966, Charnov 1976, Bowers and Breland 1996). Patch richness is, in
part, determined by microhabitat characteristics that indicate predation risk
(Thorson et al. 1998, Lima and Dill 1990). If foragers found the canopy and
open microhabitats to be equally profitable, this implies one of three things:
(1) seed predators’ natural predators are lacking or at very low numbers
at the experimental site, (2) differences in microhabitat (open vs. canopy)
are inaccurate indicators of predation risk, and/or (3) seed predators are
incapable of using microhabitat differences to assess predation risk. These
conclusions are nevertheless limited by the small sample size of the GUD
experiment and the rarity of consumption events. An alternative explanation
of the results is that the experiment was not of large enough scale to detect
how differences in microhabitat characteristics affect foraging behavior. It
is also possible that consumption events were rare because the artificial
patches could have been perceived as resource-poor, relative to the natural
O. floribunda seed patches present nearby. This is likely the case, considering
how agouti mated pairs generally occupy areas of about 10,000-
20,000m2 for foraging (Smythe 1978). Since foragers should concentrate
their foraging efforts on rich patches (MacArthur and Pianka 1966, Krebs et
al. 1974, Charnov 1976), creating poor artificial patches makes it more difficult
for effects such as of microhabitat and seed treatment to be detected.
Determining how canopy gaps affect O. floribunda seed removal
is crucial because, as Wenny and Levey (1998) showed, three-wattled
bellbirds have a tendency to regurgitate their seeds into canopy gaps. Dispersal
by three-wattled bellbirds in the lower montane rainforest resulted
in higher seedling survival rates than dispersal by the other birds, as a
result of lower fungal infection rates and favorable growth conditions available
in canopy gaps (Wenny and Levey 1998). Sites at lower altitudes that
experience significant seed removal by agoutis may have lower seedling
survival rates, both in canopy gaps and under canopy cover, but one of
these microhabitats could experience higher seed removal rates than the
other as a result of differences in patch richness, as assessed by agoutis.
Seed treatment had no influence on O. floribunda seed removal.
These results suggest that to the foragers that removed them, regurgitated
seeds are equally valuable to seeds manually removed from the pericarp,
though once again sample size is a significant limiting factor. It is also worth
noting, however, that food selection is but the second step in a forager’s
two-step diet selection process; first comes patch selection (Brown and
Morgan 1995). While the value of different seed treatments might be equal
to the foragers, it cannot yet be discarded that the foragers selected these
patches because they were able to track down a smell unique to regurgitated
seeds. To test this hypothesis, a GUD experiment where regurgitated
seeds and seeds manually removed from the pericarp are placed at
different sites of equivalent microhabitat characteristics, would be useful.
Similarly, it might also be of interest to test seeds that were dispersed by
different species to evaluate how primary dispersal mode affects the likelihood
of seed removal, post-primary dispersal. If the regurgitated state is
equally valuable to seeds with manually removed pericarps, and foragers
are able to find both at equal rates, this implies that regurgitation of O.
floribunda seeds by the primary dispersers is not of relative importance
to foragers. This conclusion would be particularly puzzling considering no
fruit consumption was observed in the duration of this experiment. After all,
the pericarp of O. floribunda can be easily removed by hand and caviomorphs
should deftly be able to access the seed inside, if they so desired.
Other than seed infestation rates (Fig. 10), there were no observable
differences among the seed treatments used. It is likely that the
higher infestation rate seen in regurgitated seeds in both experiments is a
consequence of how long these seeds were exposed to the environment
without their pericarp (considering they were collected in this state). Alternatively,
the digestive enzymes present in the primary disperser species’
guts might weaken the defenses O. floribunda seeds have against infestation
by arthropods. Either way, given that there are no other known ways
by which the pericarp is removed, and given how the pericarp must be
removed for germination to occur (Wenny 2000), the cost O. floribunda
seeds must accept, in exchange for dispersal by regurgitation, is a high infestation
rate. Learning whether or not agoutis have a preference for infested
or uninfested seeds will determine if the effects of arthropods and agoutis
on seed removal are additive. If agoutis preferentially consume infested
seeds, as gray squirrels (S. carolinensis) do (Steele et al. 1996), then seed
consumption by agoutis should not have a significant impact on Lauraceae
seedling establishment. The opposite is true if agoutis prefer uninfested
seeds. It is also possible that agoutis have a partial preference for either
treatment, are unable to determine infestation, or have no preference at
all. To evaluate seed preferences and the optimal switching point between
seed treatments, seeds could first be classified into uninfested and infested
classes via x-ray techniques (Semel and Anderson 1988) before being presented
to agoutis (in periods short enough to prevent further infestation), in
monitored adjacent patches. Furthermore, to evaluate partial preference,
this experiment could also vary the density of seed treatments and/or place
them closer to refuge, following Brown and Morgan’s (1995) methodology.
By investigating treatment preferences, we can learn more about the impact
agoutis (D. punctata) have on O. floribunda seedling establishment.
Members of the Lauraceae play particularly important roles in
restoration efforts in Monteverde because of the threatened and endangered
dispersers that depend on their fruits (Wheelwright 1991, Wenny
2000, Nadkarni and Wheelwright 2000) and because of their potential to
serve as regeneration nuclei in abandoned pastures (Howe et al. 2010). In
my study, I confirm that agoutis function as seed predators for O. floribunda.
It is also confirmed that a fraction of these seeds are cached. Equal ratios
of uninfested to infested buried seeds were found, but it is not possible
to determine if infestation occurred prior to burial, however unlikely. Given
the limitations in the GUD and seed tagging experiments, conclusions
drawn from microhabitat and seed treatment differences, as well as from
seed removal intensity, are preliminary. Replication of these experiments
with a larger sample size, as has already been elaborated, would help
elucidate the impact agoutis (and other possible foragers) have on Lauraceae
seed removal, as well as provide insight into the foraging strategies
and behavior these caviomorphs employ under different conditions. How
efficient these foragers are, how they respond to habitat heterogeneity, and
their population size, will invariably impact the distribution of O. floribunda
(and other Lauraceaous species) seeds and seedling establishment.
While it is premature to declare this impact as detrimental or beneficial to
O. floribunda, it is evident that the interaction between these two species
merits further investigation, particularly to determine whether or not agoutis
should be excluded from abandoned pastures undergoing restoration.
Having this information and applying the correct decision could enhance
the potential Lauraceae species have to function as regeneration nuclei,
and thus facilitate the reconnection of forest fragments in Monteverde.
Monteverde is just one example of a site in need of facilitated
restoration. Tropical dry forests alone, as a direct result of extensive deforestation
that took off in the 1950s, have been reduced to 1.7% of their
original expanse (Calvo-Alvarado et al. 2009), giving this biome the rank
of third highest in gross forest cover lost in the world (Hansen et al. 2010).
Ranking second, are the tropical wet forests (Hansen et al. 2010), which
exclutogether
with tropical dry forests are home to a myriad of endemic species,
now in danger of extinction (Myers et al. 2000). While deforestation
is ongoing, reforestation efforts in the tropics have increased in the recent
decades (Klugman 2011, Aide et al. 2013), particularly in Costa Rica (Calvo-
Alvarado et al. 2009), and attempts to regenerate forests through passive
restoration, protection from fire and cattle, native species enrichment,
removal of invasive grasses, and conversion of abandoned pastures to
plantations have followed. Each of these methods has met with variable
success (Griscom and Ashton 2011). It has become increasingly evident
that most important to tropical forest regeneration and reconnection is the
establishment of tree plantations protected from fire and cattle (Griscom
and Ashton 2011). These plantations must have a highly diverse assemblage
of native species (Rodrigues et al. 2011) primarily shade-intolerant
late-successionals that can shade out invasive grasses (Griscom and Ashton
2011), are animal-dispersed, and can thus function as regeneration
nuclei (Cole et al. 2010, Howe et al. 2010, Holl et al. 2011). Connecting
the fragmented forests of Central America will increase total available
habitat, help reconnect isolated populations, and protect tropical birds
throughout their migratory routes (Powell and Bjork 1995, 2004, Nadkarni
and Wheelwright 2000), thereby protecting the biodiversity of the tropics.
Yet, tropical forests are not the only biomes under threat by habitat loss
and deforestation; North America and Asia have the highest rates of gross
forest cover loss in the world, together accounting for 52.9% of global forest
cover loss in 2000–2005 (Hansen et al. 2010). Species loss around
the globe has led some scientists to claim that our actions have brought
our planet to the dawn of the sixth mass extinction (Barnosky et al. 2011).
Given the high levels of biodiversity in tropical environments, restoring and
reconnecting tropical forests is of high priority, but ultimately the mission
to protect biodiversity is global (Myers et al. 2000). In order to carry out
this task, more ecological studies are needed to elucidate how to properly
accelerate healthy restoration in different biomes. Our ability to identify
the conditions necessary for successful reforestation will determine whether
or not the current rate of faunal extinction will continue to accelerate
(Myers et al. 2000). Expanding on what we already know about foraging
behavior, seed dispersal, seedling establishment, plant demography,
and regeneration nuclei will prove indispensable as we continue to strive
for the conservation of endangered species and ecosystems worldwide.
Acknowledgements
Without my friends, Tamara Kuehne, Ashley Gora, Laura Cussen,
Jacob Simmonds, Marike Louw, Lana Panitch, Abby Brownell, Beth
Herbert, Tatjana Stooß, Merlin Sheldrake, Ian Iyengar, William Beltrán,
Kirk Geier, Maricella Solis, Anya Kogan, Diego Ormeño, Shashikala
Wanigasinghe, Randy Chinchilla, Ryan Spanier, Alyssa Conway, Shevi
Wosk, Kayla Thieken, Joaquin Basile, Arelí Tejada, Elizabeth Bulley,
Linda Strauss, Mao Medina, Yoko Hama, Zhiyu Deng, and Analí Vargas,
I would have accomplished nothing. Likewise, without the support
of my wonderful family, the guidance and kindness of Dr. Lynn Westley,
Dr. Sean Menke, and Dr. Glenn Adelson as my thesis committee, the
professors of the Biology and Environmental Studies Departments at
Lake Forest College, Dr. Frank’s assistance with statistics, the lessons
taught to me by the staff of the Monteverde Institute, and the incredible
experiences and adventures I shared with many great minds at Barro
Colorado Island, I would not be the person I am today – thank you all!
Last but not least, I would like to thank the Grace E. Groner Foundation
for believing in my potential and providing me with funding for three fully
enriching internships, the last of which allowed me to conduct this research.
References
Abu Baker, M. A., and J. S. Brown. 2009. Patch area, substrate depth, and richness
affect giving‐up densities: a test with mourning doves and cottontail rabbits.
Oikos 118:1721-1731.
Abu Baker, M. A. A., and J. S. Brown. 2014. Foraging in space and time structure an
African small mammal community. Oecologia 175:521-535.
Aide, T. M., M. L. Clark, H. R. Grau, D. López‐Carr, M. A. Levy, D. Redo, M. Bonilla‐
Moheno, G. Riner, M. J. Andrade‐Núñez, and M. Muñiz. 2013. Deforestation
and reforestation of Latin America and the Caribbean (2001–2010). Biotropica
45:262-271.
Barnett, R. J. 1977. The effect of burial by squirrels on germination and survival of oak
and hickory nuts. American Midland Naturalist :319-330.
Barnosky, A. D., N. Matzke, S. Tomiya, G. O. Wogan, B. Swartz, T. B. Quental, C. Marshall,
J. L. McGuire, E. L. Lindsey, and K. C. Maguire. 2011. Has the Earth’s
sixth mass extinction already arrived? Nature 471:51-57.
Bell, T., R. P. Freckleton, and O. T. Lewis. 2006. Plant pathogens drive density‐dependent
seedling mortality in a tropical tree. Ecology Letters 9:569-574.
Berger-Tal, O., K. Embar, B. P. Kotler, and D. Saltz. 2015. Everybody loses: intraspecific
competition induces tragedy of the commons in Allenby’s gerbils. Ecology
96:54-61.
Bowers, M., J. Jefferson, and M. Kuebler. 1993. Variation in giving-up densities of
foraging chipmunks (Tamias striatus) and squirrels (Sciurus carolinensis). Oikos
66:229-236.
Bowers, M. A., and B. Breland. 1996. Foraging of gray squirrels on an urban-rural
gradient: use of the GUD to assess anthropogenic impact. Ecological Applications
6:1135-1142.
Brotherton, P., T. Clutton-Brock, M. O’Riain, D. Gaynor, L. Sharpe, R. Kansky, and G.
McIlrath. 2001. Offspring food allocation by parents and helpers in a cooperative
mammal. Behavioral Ecology 12:590-599.
Brown, J. S. 1988. Patch use as an indicator of habitat preference, predation risk, and
competition. Behavioral Ecology and Sociobiology 22:37-47.
Brown, J.S., R. A. Morgan, and B. D. Dow. 1992. Patch use under predation risk: II.
A test with fox squirrels, Sciurus niger. Annales Zoologici Fennici 29:311-318.
Brown, J. S., and R. A. Morgan. 1995. Effects of foraging behavior and spatial scale
on diet selectivity: a test with fox squirrels. Oikos 74:122-136.
Brown, J. S., B. P. Kotler, and W. A. Mitchell. 1997. Competition between birds and
mammals: a comparison of giving-up densities between crested larks and
gerbils. Evolutionary Ecology 11:757-771.
Calvo-Alvarado, J., B. McLennan, A. Sánchez-Azofeifa, and T. Garvin. 2009. Deforestation
and forest restoration in Guanacaste, Costa Rica: putting conservation
policies in context. Forest Ecology and Management 258:931-940.
Caraco, T. 1983. White-crowned sparrows (Zonotrichia leucophrys): foraging preferences
in a risky environment. Behavioral Ecology and Sociobiology 12:63-69.
Caraco, T. 1982. Aspects of risk-aversion in foraging white-crowned sparrows. Animal
Behaviour 30:719-727.
Carson, W. P., J. T. Anderson, E. Leigh, and S. A. Schnitzer. 2008. Challenges associated
with testing and falsifying the Janzen-Connell hypothesis: a review and
critique. Pages 210-241 in W. P Carson and S. A. Schnitzer, editors. Tropical
forest community ecology. Wiley-Blackwell Publishing, Oxford, UK
Charnov, E. L. 1976. Optimal foraging, the marginal value theorem. Theoretical Population
Biology 9:129-136.
Chinchilla, R. 2015. Determinación del uso de la tierra y la fragmentación boscosa
en el Corredor Biológico Pájaro Campana: promoviendo estrategias de
conservación y manejo integral. Práctica Dirigida para optar por el grado de
Licenciatura en Geografía. Escuela de Geografía, Universidad de Costa Rica.
Clark, D. A., and D. B. Clark. 1984. Spacing dynamics of a tropical rain forest tree:
evaluation of the Janzen-Connell model. American Naturalist 124:769-788.
Clark, D. A., and D. B. Clark. 1981. Effects of seed dispersal by animals on the regeneration
of Bursera graveolens (Burseraceae) on Santa Fe Island, Galapagos.
Oecologia 49:73-75.
Cole, R. J., K. D. Holl, and R. A. Zahawi. 2010. Seed rain under tree islands planted
to restore degraded lands in a tropical agricultural landscape. Ecological Applications
20:1255-1269.
Comita, L. S., S. A. Queenborough, S. J. Murphy, J. L. Eck, K. Xu, M. Krishnadas,
N. Beckman, and Y. Zhu. 2014. Testing predictions of the Janzen–Connell
hypothesis: a meta‐analysis of experimental evidence for distance‐and density‐
dependent seed and seedling survival. Journal of Ecology 102:845-856.
Connell, J. H. 1971. On the role of natural enemies in preventing competitive exclutogether
sion in some marine animals and in rain forest trees. Pages 298–312 in P. J.
den Boer, and G. R. Gradwell, editors. Dynamics of populations. Centre for
Agricultural Publishing and Documentation, Wageningen, The Netherlands.
Croze, H. 1970. Searching image in carrion crows: hunting strategy in a predator
and some anti-predator devices in camouflaged prey. Parey, Berlin, Germany.
Dalling, J. W., A. S. Davis, B. J. Schutte, and A. Elizabeth Arnold. 2011. Seed survival
in soil: interacting effects of predation, dormancy and the soil microbial community.
Journal of Ecology 99:89-95.
Dalling, J., H. Muller‐Landau, S. Wright, and S. Hubbell. 2002. Role of dispersal in
the recruitment limitation of neotropical pioneer species. Journal of Ecology
90:714-727.
De la Peña-Domene, M., C. Martínez-Garza, and H. F. Howe. 2013. Early recruitment
dynamics in tropical restoration. Ecological Applications 23:1124-1134.
De la Peña-Domene, M., C. Martínez-Garza, S. Palmas-Pérez, E. Rivas-Alonso, and
H. F. Howe. 2014. Roles of birds and bats in early tropical-forest restoration.
PloS one 9:e104656.
Dixon, M. D., W. C. Johnson, and C. S. Adkisson. 1997. Effects of weevil larvae on
acorn use by blue jays. Oecologia 111:201-208.
Doniol-Valcroze, T., V. Lesage, J. Giard, and R. Michaud. 2011. Optimal foraging
theory predicts diving and feeding strategies of the largest marine predator.
Behavioral Ecology 22:880-888.
Emerson, S. E., and J. S. Brown. 2012. Using giving-up densities to test for dietary
preferences in primates: an example with samango monkeys (Cercopithecus
(nictitans) mitis erythrarchus). International Journal of Primatology 33:1420-
1438.
FCC (Fundación Conservacionista Costarricense). [no date]. Forever forest: Creating
and restoring migratory bird refuges in Costa Rica. http://lasi.lynchburg.edu/
shahady_t/public/BOSQUE.pdf.
Forget, P. 1997. Effect of microhabitat on seed fate and seedling performance in
two rodent-dispersed tree species in rain forest in French Guiana. Journal of
Ecology 85:693-703.
Forget, P. 1996. Removal of seeds of Carapa procera (Meliaceae) by rodents and
their fate in rainforest in French Guiana. Journal of Tropical Ecology 12:751-
761.
Forget, P. 1993. Post-dispersal predation and scatterhoarding of Dipteryx panamensis
(Papilionaceae) seeds by rodents in Panama. Oecologia 94:255-261.
Forget, P. 1992. Seed removal and seed fate in Gustavia superba (Lecythidaceae).
Biotropica 24:408-414
Forget, P. 1990. Seed-dispersal of Vouacapoua americana (Caesalpiniaceae) by caviomorph
rodents in French Guiana. Journal of Tropical Ecology 6:459-468.
Forget, P., and T. Milleron. 1991. Evidence for secondary seed dispersal by rodents in
Panama. Oecologia 87:596-599.
Fox, J. 1982. Adaptation of gray squirrel behavior to autumn germination by white oak
acorns. Evolution 36:800-809.
Gallery, R. E., J. W. Dalling, and A. E. Arnold. 2007. Diversity, host affinity, and distribution
of seed-infecting fungi: a case study with Cecropia. Ecology 88:582-
588.
Gallery, R. E., D. J. Moore, and J. W. Dalling. 2010. Interspecific variation in susceptibility
to fungal pathogens in seeds of 10 tree species in the neotropical genus
Cecropia. Journal of Ecology 98:147-155.
Golley, F. B., J. T. McGinnis, R. G. Clements, G. Child, and M. Duever. 1969. The
structure of tropical forests in Panama and Colombia. Bioscience 19:693-696.
Gorchov, D. L., F. Cornejo, C. Ascorra, and M. Jaramillo. 1993. The role of seed
dispersal in the natural regeneration of rain forest after strip-cutting in the
Peruvian Amazon. Vegetatio 107:339-349.
Green, R. F. 1979. Functional response and the effect of a Bayesian predator on prey
distribution. Technical Report No. 56. http://www.d.umn.edu/~rgreen/TR%20
No.%2056.pdf.
Griscom, H. P., and M. S. Ashton. 2011. Restoration of dry tropical forests in Central
America: a review of pattern and process. Forest Ecology and Management
261:1564-1579.
Halliday, W. D., and D. W. Morris. 2013. Safety from predators or competitors? Interference
competition leads to apparent predation risk. Journal of Mammalogy
94:1380-1392.
Hansen, M. C., S. V. Stehman, and P. V. Potapov. 2010. Quantification of global gross
forest cover loss. Proceedings of the National Academy of Sciences of the
United States of America 107:8650-8655.
Hayashi, M., K. L. Feilich, and D. J. Ellerby. 2009. The mechanics of explosive seed
dispersal in orange jewelweed (Impatiens capensis). Journal of Experimental
Botany 60:2045-2053.
Herrera, C. M. 1995. Plant-vertebrate seed dispersal systems in the Mediterranean:
ecological, evolutionary, and historical determinants. Annual Review of Ecology
and Systematics 26:705-727.
Herrera, C. M., P. Jordano, L. Lopez-Soria, and J. A. Amat. 1994. Recruitment of
a mast-fruiting, bird-dispersed tree: bridging frugivore activity and seedling
establishment. Ecological Monographs 64:315-344.
Holdridge, L. R. 1967. Life zone ecology. Tropical Science Center, San José, Costa
Rica.
Holl, K. D., R. A. Zahawi, R. J. Cole, R. Ostertag, and S. Cordell. 2011. Planting
seedlings in tree islands versus plantations as a large‐scale tropical forest
restoration strategy. Restoration Ecology 19:470-479.
Holt, R. D. 1977. Predation, apparent competition, and the structure of prey communities.
Theoretical Population Biology 12:197-229.
Howe, H. F. 1979. Fear and frugivory. American Naturalist 114:925-931.
Howe, H. F. 1989. Scatter-and clump-dispersal and seedling demography: hypothesis
and implications. Oecologia 79:417-426.
Howe, H. F., and M. N. Miriti. 2004. When seed dispersal matters. Bioscience 54:651-
660.
Howe, H. F., and J. Smallwood. 1982. Ecology of seed dispersal. Annual Review of
Ecology and Systematics 13:201-228.
Howe, H. F., Y. Urincho-Pantaleon, M. de la Peña-Domene, and C. Martínez-Garza.
2010. Early seed fall and seedling emergence: precursors to tropical restoration.
Oecologia 164:731-740.
Howe, H., E. W. Schupp, and L. Westley. 1985. Early consequences of seed dispersal
for a neotropical tree (Virola surinamensis). Ecology 66:781-791.
Hubbell, S. P. 1980. Seed predation and the coexistence of tree species in tropical
forests. Oikos 35:214-229.
Hubbell, S. P. 1979. Tree dispersion, abundance, and diversity in a tropical dry forest.
Science (New York, NY) 203:1299-1309.
Iwasa, Y., M. Higashi, and N. Yamamura. 1981. Prey distribution as a factor determining
the choice of optimal foraging strategy. American Naturalist 117:710-723.
Jacob, J., and J. S. Brown. 2000. Microhabitat use, giving‐up densities and temporal
activity as short‐and long‐term anti‐predator behaviors in common voles.
Oikos 91:131-138.
Jacobs, L. F., and E. R. Liman. 1991. Grey squirrels remember the locations of buried
nuts. Animal Behaviour 41:103-110.
Jansen, P. A., and P. Forget. 2001. Scatterhoarding rodents and tree regeneration.
Pages 275-288 in F. Bongers, P. Charles-Dominique, P. Forget, M. Théry,
editors. Dynamics and plant-animal interactions in a Neotropical rainforest,
Kluwer Academic Publishers, Netherlands.
Janson, C. H. 1983. Adaptation of fruit morphology to dispersal agents in a neotropical
forest. Science (New York, NY) 219:187-189.
Janzen, D. H. 1988. Management of habitat fragments in a tropical dry forest: growth.
Annals of the Missouri Botanical Garden :105-116.
Janzen, D. H. 1970. Herbivores and the number of tree species in tropical forests.
American Naturalist 104:501-528.
John, R., J. W. Dalling, K. E. Harms, J. B. Yavitt, R. F. Stallard, M. Mirabello, S. P. Hubbell,
R. Valencia, H. Navarrete, M. Vallejo, and R. B. Foster. 2007. Soil nutrients
influence spatial distributions of tropical tree species. Proceedings of the
National Academy of Sciences of the United States of America 104:864-869.
Klugman, J. 2011. Human Development Report 2011. Sustainability and equity: a
better future for all. Sustainability and equity: a better future for all (November
2, 2011). UNDP-HDRO Human Development Reports.
Kotler, B. P., J. S. Brown, S. R. Dall, S. Gresser, D. Ganey, and A. Bouskila. 2002.
Foraging games between gerbils and their predators: temporal dynamics of
resource depletion and apprehension in gerbils. Evolutionary Ecology Research
4:495-518.
Krebs, J. R., J. C. Ryan, and E. L. Charnov. 1974. Hunting by expectation or optimal
foraging? A study of patch use by chickadees. Animal Behaviour 22:953-IN3.
Leigh Jr, E. G. 1999. Tropical Forest Ecology: A View from Barro Colorado Island.
Oxford University Press, New York, USA.
Lima, S. L. 1993. Ecological and evolutionary perspectives on escape from predatory
attack: a survey of North American birds. The Wilson Bulletin 105:1-47.
Lima, S. L. 1988. Vigilance during the initiation of daily feeding in dark-eyed juncos.
Oikos 53:12-16.
Lima, S. L., and L. M. Dill. 1990. Behavioral decisions made under the risk of predation:
a review and prospectus. Canadian Journal of Zoology 68:619-640.
Lima, S. L., T. J. Valone, and T. Caraco. 1985. Foraging-efficiency-predation-risk
trade-off in the grey squirrel. Animal Behaviour 33:155-165.
Lisa, L. 2012. Vegetation biodiversity survey and seed rain assessment as part of
long-term reforestation monitoring at the Cloud Forest School in Monteverde,
Costa Rica. Master of Science thesis, Central European University, Budapest.
MacArthur, R. H., and E. R. Pianka. 1966. On optimal use of a patchy environment.
American Naturalist 100:603-609.
MacArthur, R., and R. Levins. 1964. Competition, habitat selection, and character
displacement in a patchy environment. Proceedings of the National Academy
of Sciences of the United States of America 51:1207-1210.
Mangan, S. A., S. A. Schnitzer, E. A. Herre, K. M. Mack, M. C. Valencia, E. I. Sanchez,
and J. D. Bever. 2010. Negative plant-soil feedback predicts tree-species relative
abundance in a tropical forest. Nature 466:752-755.
McNair, J. N. 1982. Optimal giving-up times and the marginal value theorem. American
Naturalist 119:511-529.
Miller, A. P. 2012. Ecotourism Development in Costa Rica: The Search for Oro Verde.
Lexington Books, New York, USA.
MINAE (Ministerio del Ambiente y Energía) and SINAC (Sistema Nacional de Areas
de Conservación Costa Rica). 2003. Informe Nacional sobre el Sistema de
Areas Silvestres Protegidas. http://www.inbio.ac.cr/es/biod/Resumen.pdf.
Molokwu, M. N., J. Nilsson, and O. Olsson. 2011. Diet selection in birds: trade-off
between energetic content and digestibility of seeds. Behavioral Ecology
3:639-647.
Mori, Y. 1998. Optimal choice of foraging depth in divers. Journal of Zoology 245:279-
283.
Myers, N., and R. Tucker. 1987. Deforestation in Central America: Spanish Legacy
and North American Consumers. Environmental Review: ER 11:55-71.
Myers, N., R. A. Mittermeier, C. G. Mittermeier, G. A. Da Fonseca, and J. Kent. 2000.
Biodiversity hotspots for conservation priorities. Nature 403:853-858.
Nadkarni, N. M., and N. T. Wheelwright. 2000. Monteverde: Ecology and Conservation
of a Tropical Cloud Forest. Oxford University Press, New York, USA.
Nathan, R., G. G. Katul, H. S. Horn, S. M. Thomas, R. Oren, R. Avissar, S. W. Pacala,
and S. A. Levin. 2002. Mechanisms of long-distance dispersal of seeds by
wind. Nature 418:409-413.
Newman, J. A. 1991. Patch use under predation hazard: foraging behavior in a simple
stochastic environment. Oikos 61:29-44.
Noble, J. C. 1975. The effects of emus (Dromaius novaehollandiae Latham) on the
distribution of the nitre bush (Nitraria billardieri DC.). Journal of Ecology
63:979-984.
Packer, A., and K. Clay. 2000. Soil pathogens and spatial patterns of seedling mortality
in a temperate tree. Nature 404:278-281.
Pianka, E. R. 1969. Sympatry of desert lizards (Ctenotus) in Western Australia. Ecology
50:1012-1030.
Pierce, G., and J. Ollason. 1987. Eight reasons why optimal foraging theory is a complete
waste of time. Oikos 49:111-118.
Post, D. M. 1992. Change in nutrient content of foods stored by eastern woodrats
(Neotoma floridana). Journal of Mammalogy 73:835-839.
Powell, G. V., and R. D. Bjork. 2004. Habitat linkages and the conservation of tropical
biodiversity as indicated by seasonal migrations of three‐wattled bellbirds.
Conservation Biology 18:500-509.
Powell, G. V., and R. Bjork. 1995. Implications of intratropical migration on reserve
design: a case study using Pharomachrus mocinno. Conservation Biology
9:354-362.
Pulliam, H. R. 1974. On the theory of optimal diets. American Naturalist 108:59-74.
Pulliam, H. R. 1975. Diet optimization with nutrient constraints. American Naturalist
109:765-768.
Pyke, G. H. 1984. Optimal foraging theory: a critical review. Annual Review of Ecology
and Systematics 15:523-575.
R Development Core Team. 2012. R: A language and environment for statistical
computing. R Foundation for statistical computing, Vienna, Austria. http://www.
R-project.org
Raich, J. W., and G. W. Khoon. 1990. Effects of canopy openings on tree seed germination
in a Malaysian dipterocarp forest. Journal of Tropical Ecology 6:203-
217.
Riginos, C. 2015. Climate and the landscape of fear in an African savanna. Journal of
Animal Ecology 84:124-133.
Rodrigues, R. R., S. Gandolfi, A. G. Nave, J. Aronson, T. E. Barreto, C. Y. Vidal, and P.
H. Brancalion. 2011. Large-scale ecological restoration of high-diversity tropical
forests in SE Brazil. Forest Ecology and Management 261:1605-1613.
Rojas, L. A., and M. I. Chavarría. 2005. Corredores Biológicos de Costa Rica. Corredor
Biológico Mesoamericano sección Costa Rica. San José, Costa Rica.
Rosenzweig, M. L., and P. W. Sterner. 1970. Population ecology of desert rodent communities:
body size and seed-husking as bases for heteromyid coexistence.
Ecology 51:217-224.
Royama, T. 1970. Factors governing the hunting behaviour and selection of food by
the great tit (Parus major L.). The Journal of Animal Ecology 39:619-668.
Royama, T. 1971. A comparative study of models for predation and parasitism. Researches
on Population Ecology 13:1-91.
Ryan, K. A. 2012. Bellbird Biological Corridor Water Monitoring Program: Objectives,
Current State, and Future Directions. http://lasi.lynchburg.edu/shahady_t/
public/Costa%20Rica%20Watershed%20Research.pdf
Sánchez-Azofeifa, G. A., R. C. Harriss, and D. L. Skole. 2001. Deforestation in Costa
Rica: A quantitative analysis using remote sensing imagery. Biotropica
33:378-384.
Schoener, T. W. 1974. The compression hypothesis and temporal resource partitioning.
Proceedings of the National Academy of Sciences of the United States of
America 71:4169-4172.
Schupp, E. W., and M. Fuentes. 1995. Spatial patterns of seed dispersal and the
unification of plant population ecology. Ecoscience 2:267-275.
Semel, B., and D. C. Andersen. 1988. Vulnerability of acorn weevils (Coleoptera: Curculionidae)
and attractiveness of weevils and infested Quercus alba acorns to
Peromyscus leucopus and Blarina brevicauda. American Midland Naturalist
119:385-393.
Smythe, N. 1978. The natural history of the central American agouti. Smithsonian
Contributions to Zoology 257:1-52.
Spencer, E. E., M. S. Crowther, and C. R. Dickman. 2014. Risky business: do native
rodents use habitat and odor cues to manage predation risk in Australian
deserts? PloS one 9:e90566.
Staniland, I. J., N. Gales, N. L. Warren, S. L. Robinson, S. Goldsworthy, and R.
Casper. 2010. Geographical variation in the behaviour of a central place forager:
Antarctic fur seals foraging in contrasting environments. Marine Biology
157:2383-2396.
Stapanian, M. A., and C. C. Smith. 1984. Density-dependent survival of scatterhoarded
Nuts: an experimental approach. Ecology 65:1387-1396.
Steele, M. A., L. Z. Hadj-Chikh, and J. Hazeltine. 1996. Caching and feeding decisions
by Sciurus carolinensis: responses to weevil-infested acorns. Journal
of Mammalogy 77:305-314.
Stephens, P. A., C. Carbone, I. L. Boyd, J. M. McNamara, K. C. Harding, and A. I.
Houston. 2008. The scaling of diving time budgets: insights from an optimality
approach. The American Naturalist 171:305-314.
Swamy, V., and J. W. Terborgh. 2010. Distance‐responsive natural enemies strongly
influence seedling establishment patterns of multiple species in an Amazonian
rain forest. Journal of Ecology 98:1096-1107.
Thomas, D., D. Cloutier, M. Provencher, and C. Houle. 1988. The shape of bird-and
bat-generated seed shadows around a tropical fruiting tree. Forma del banco
de semillas alrededor de un árbol tropical en fructificación generada por aves
y murciélagos. Biotropica 20:347-348.
Thorson, J. M., R. A. Morgan, J. S. Brown, and J. E. Norman. 1998. Direct and indirect
cues of predatory risk and patch use by fox squirrels and thirteen-lined ground
squirrels. Behavioral Ecology 9:151-157.
Traveset, A., R. Heleno, and M. Nogales. 2014. The ecology of seed dispersal. Pages
62-93 in R. S. Gallagher, editor. Seeds: The ecology of regeneration in plant
communities. CAB International, Massachussets, USA.
Valone, T. J., and J. S. Brown. 1989. Measuring patch assessment abilities of desert
granivores. Ecology 70:1800-1810.
Vander Wall, S. B., and R. P. Balda. 1977. Coadaptations of the Clark’s nutcracker
and the pinon pine for efficient seed harvest and dispersal. Ecological Monographs
47:89-111.
Vargas, A. 2007. País quiere ser primera nación con balance neutron de carbon. La
Nación 21 February. http://www.nacion.com/vivir/Pais-primera-balance-neutro-
carbono_0_886711364.html
Wenny, D. G. 2000. Seed dispersal, seed predation, and seedling recruitment of a
neotropical montane tree. Ecological Monographs 70:331-351.
Wenny, D. G. 2005. Post-dispersal seed fate of some cloud forest tree species in
Costa Rica. Pages 351-363 in Lambert, J. E., P. E. Hulme, and S. B. Vander
Wall editors. Seed Fate: Predation, Dispersal, and Seedling Establishment.
CAB International, Massachusetts, USA.
Wenny, D. G., and D. J. Levey. 1998. Directed seed dispersal by bellbirds in a tropical
cloud forest. Proceedings of the National Academy of Sciences of the United
States of America 95:6204-6207.
Wheelwright, N. T. 1991. How long do fruit-eating birds stay in the plants where they
feed? Biotropica 23:29-40.
Wheelwright, N. T., W. A. Haber, K. G. Murray, and C. Guindon. 1984. Tropical
fruit-eating birds and their food plants: a survey of a Costa Rican lower montane
forest. Biotropica 16:173-192.
Willson, M. 1993. Dispersal mode, seed shadows, and colonization patterns. Vegetatio
107:261-280.
Wright, S. J. 1983. The dispersion of eggs by a bruchid beetle among Scheelea palm
seeds and the effect of distance to the parent palm. Ecology 64:1016-1021.
Wunderle Jr, J. M. 1997. The role of animal seed dispersal in accelerating native
forest regeneration on degraded tropical lands. Forest Ecology and Management
99:223-235.
Yarranton, G., and R. Morrison. 1974. Spatial dynamics of a primary succession:
nucleation. Journal of Ecology 62:417-428.