Firings of a Schizophrenic Mind: Faulty neurotransmission and genetics
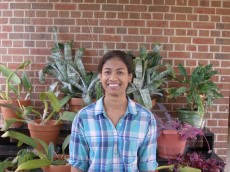
Madhavi Senagolage and Peter Sullivan
Department of Biology
Lake Forest College
Lake Forest, Illinois 60045
Download PDF
Abstract
Schizophrenia is a common neurological disorder which affects 1.0% of the world population and causes a combination of positive (hallucinations, delusions), negative (anhedonia), and cognitive symptoms. Neuropathology is currently explained by genetics and the dopamine, glutamate, and GABA neurotransmitter theories. The dopamine (DA) hypothesis implicates that hyperactive dopaminergic systems lead to schizophrenia, while compromised DA regulation mimics positive symptoms of schizophrenia. The Glutamate and GABA hypotheses explain the negative and cognitive symptoms of schizophrenia left unexplained by the dopamine hypothesis. Mutations in NMDA-receptor subunits, NR1 and NR2A, are main components of regulating glutamate, while the enzyme GAD regulates GABA neurotransmission. Additionally, several genes, including DISC1, DTNBP1, and NRG3, among many possible de novo copy number variant (CNV) mutations, increase susceptibility to schizophrenia. DISC1 regulates mitochondrial function through mitofilin, while NRG3 influences migration and patterning during neurodevelopment through the production of isoforms. The large number of de novo CNV mutations may also contribute to schizophrenia although individual CNV mutations are rare. While interacting neurotransmitter system and genetic alterations can lead to schizophrenia, further evidence is required to understand the interactions of these systems and provide insight into a complex neurological disorder lacking a clear source or pathology.
Introduction
Schizophrenia is a complex neurological disease affecting about 0.5-1% of the world’s population and creating social, economic, and health concerns (Park et al. 2010). Schizophrenia mainly affects the dorsolateral prefrontal cortex, cerebellum and striatum of the brain. Since being first diagnosed in the 19th century, the symptoms of schizophrenia have been grouped into three divisions: positive, negative, and cognitive. Positive symptoms are not common to the general population, but are found in the disorder, while negative symptoms are usually found in the general population, but not in the disorder. The positive symptoms of schizophrenia include hallucinations and delusions. The negative symptoms include apathy, anhedonia, and withdrawal. Cognitive symptoms include attention, working memory and executive functions are affected (Hashimoto, 2003). Symptoms usually appear between sixteen and thirty years of age and onset is rarely seen in those over forty-five years old. Schizophrenia is common worldwide and occurs equally among race and gender. While schizophrenia is not overrepresented in any gender or ethnicity, men tend to develop symptoms earlier, and negative symptoms are usually detected before any positive or cognitive symptoms.
Although the symptoms of schizophrenia have been well-recorded, the causes of schizophrenia are varied and not well understood. The possible factors affecting the onset of schizophrenia include both environmental, such as the season born, childhood trauma (Tsuang and Stone 2001), and drug use (Lukoff, 1986); and genetic, such as mutations in key genes affecting neuro pathway. However, many studies suggest that an intricate relationship between genetic predisposition and environmental factors exists (Tienari et al. 2004). Just as the causes vary, the symptoms of schizophrenia also vary between cases and over time (Tandon et al., 2009). This variation makes diagnosis difficult. Diagnosis is currently only done clinically diagnosis by observing symptoms over a six month period. Also due to the mixed symptoms of schizophrenia, treatment, consisting mostly of antipsychotic drugs aimed at correcting neurotransmitter release, is difficult and generally only alleviates the positive symptoms of schizophrenia, leaving the negative and cognitive symptoms unchanged. These antipsychotics have a low tolerability and must be taken over long periods of time, which may increase the risk of detrimental side effects.
Schizophrenia is a complex disorder due to the interaction of many genes and cellular neurotransmission pathways. Although named over one-hundred years ago, the cause of schizophrenia remains unknown. Understanding the biological basis of the complex neural circuitry in schizophrenia will help to understand the fundamental nature of schizophrenia and develop new treatment and preventive strategies in the future. There are several neurotransmitter systems found to be altered in Schizophrenia, including the widely studies dopamine, glutamate, and GABA neurotransmission systems. Studies have suggested that the dopamine transport system is hyperactive, while glutamate and GABA are hypoactive in pathological conditions. In addition to these pathways playing a potential role in schizophrenia, genetic defects have also been associated with an increased susceptibility for developing schizophrenia. The most consistent genetic link occurs in the genes DISC1, NRG3, and DTNBP1 (McGuire et al, 2008). DISC1 and NRG3 both aid in neuron regulation and development, while DTNBP1 plays a role in neurotransmitter release. In addition to these specific susceptibility genes, many de novo copy number variant (CNV) mutations also occur in schizophrenia due to detrimental mircodeletions and microduplications.
The lack of a clear connection between schizophrenia and a genetic or molecular cause has led to many studies over the past decade, in attempt to elucidate possible causes of schizophrenia. These studies will look at genetic mutations in a multitude of genes and deficient neurotransmission of glutamate, dopamine, and GABA. Despite the discoveries of multiple genetic and molecular defects in schizophrenia patients, the connection as to how these changes actually cause schizophrenia are still only speculated. This paper will combine the findings of multiple research articles in attempt to further understand the connection between known genetic and molecular discrepancies and hypothesized pathways of schizophrenia. Based on these findings, we hypothesized that schizophrenia is a result of altered and integrated neurotransmitter signaling that is favored under altered genetics. In order to understand the underlying nature and causes of schizophrenia, we are focusing on three major areas of inquiry in the field: the examination of neurochemical abnormalities, the integration of neurotransmitter systems, and the identification of candidate genes associated with increased susceptibility.
Neurochemical Abnormalities
In this part of our review we will focus on understanding the abnormalities in three main underlying neurotransmitter systems: dopamine, glutamate and gamma aminobutyric acid (GABA). We will connect the major discoveries in the field that showed involvement of neurotransmitter transporters, receptors and catalytic enzymes and how alterations to these systems can result in schizophrenic symptoms.
Dopamine Neurotransmission
Of all the neurotransmitter circuits believed to be involved in schizophrenia, the dopamine theory has been the most enduring. The dopamine (DA) hypothesis suggests that hyperactivity of the dopamine circuit leads to the characteristic symptoms of schizophrenia (Van Rossum, 1966). The first insight into this theory become known when neuroleptic drugs such as chlorpromazine and haloperidol alleviated the schizophrenic symptoms by acting as antagonists for DA D2 receptor sites (Seeman et al, 1976). Soon after, increased D2 receptors in post-mortem brains of schizophrenia patients (Lee and Seeman, 1977) and increased dopamine occupancy at D2 receptors were also reported (Anissa Abi-Dargham et al, 2000).The dopamine hypothesis was further supported with the finding that drugs like amphetamine (AMPH), DA receptor stimulants, were found to cause schizophrenic symptoms in both humans and in animal models (Angrist et al, 1974; Castner et al, 1999).
Evidence that confirmed the alterations in dopamine levels in Schizophrenia led researchers to focus on other molecules that are involved in this neurochemical pathway, such as dopamine transporter (DAT) and catechol-o-methyltransferase (COMT) enzyme, both regulate dopamine activity in the synapses, but through different mechanisms. The DAT plays a role in locomotion in normal individuals by controlling the dopamine levels in the basal ganglia and nucleus accumbens (Giros et al, 1996). In 1996, Giros and his colleagues found out that mice lacking DAT (DAT-KO) were unable to re-uptake released DA (Giros et al, 1996) leading to hyperdopaminergia. These knockout mice also exhibited hyper-locomotion, which could be reversed by administration of DA receptor antagonists (Giros et al, 1996). COMT is an enzyme involved in monoamine metabolism (Williams et al, 2007) and is expressed mainly in the prefrontal cortex where it is found to be involved in dopamine metabolism. When COMT is inhibited in mice, their extracellular DA levels were found to be increased supporting the idea of possible alteration in DA activity in schizophrenic brain (Tunbridge, 2004). Recently, it was found out that frontotemporatal function during verbal function modulated by COMT is altered in Schizophrenia patients (Prata et al, 2009). A study that immediately followed found that the COMT and DAT genes interact nonadditively to regulate executive functions. This effect is altered in schizophrenia which might be leading to the abnormal hyper activity of dopamine (Prata et al, 2009).
These data support the idea that dopamine neurotransmission is altered in schizophrenia. However, the dopamine hypothesis alone does not explain all the symptoms underlying schizophrenia, suggesting that involvement of one or more neurochemical systems. With the possibility of many neurotransmitters involved in schizophrenia, the dopamine hypothesis has been modified.
Glutamate Neurotransmission
Glutamate dysfunction in schizophrenia was first discovered when phencyclidine (PCP) and ketamine were found to closely mimic Schizophrenia (Luby et al., 1959). The PCP mimics the negative symptoms of schizophrenia far better than other drug-induced psychoses such as those induced by amphetamine (Sawa and Snyden, 2002). In the late twentieth century many studies found that PCP and ketamine act as noncompetitive antagonists of NMDA receptors (Javitt and Zhukin, 1991; Ellison, 1995; Malhotra et al., 1997). Soon after, many animal models were used to understand the nature of the disease (Carlsson, 1990; Corbett et al., 1993). A study done in mice models by Mohn and colleagues further support the evidence of glutamate receptor deficiency that leads to schizophrenia. In their study, they created mice with only 5% -10% of normal levels of the essential NR1 subunit of NMDA receptor. The mice displayed increased motor activity, stereotypy, and abnormal social and sexual interactions. They also found that these symptoms could be attenuated by administering haloperidol or clozapine, an antipsychotic medication (Mohn et al, 1999). After many promising results, genes regulating glutamate neurotransmission have become prominent candidates for genetic association studies. GRM3, a metabotropic glutamate receptor-modulating synaptic glutamate is one of the promising canidates, which controls expression of EEAT, a glutamate transporter (Egan et al, 2004). The SNP4 A allele of the gene was found to be involved in poor cognitive performance. Also, in postmortem human prefrontal cortex, SNP4 AA homozygotes had lower mRNA levels of the glutamate transporter EAAT2 (Egan et al, 2004).
Together all these evidence support the idea that glutamate function is reduced in schizophrenic patients. While both dopamine and glutamate hypothesis together explained positive and negative symptoms of schizophrenia, the cognitive symptoms were left unexplained, suggesting an involvement of another neurophysiologic system.
GABA Neurotransmission
Before the GABA hypothesis of schizophrenia was proposed, the neural circuits underlying cognitive impairments such as distorted working memory and altered executive functions remained unknown to the scientific community. The GABA hypothesis states that decreased levels of GABA in neurotransmission cause cognitive impairments of schizophrenia (Cho et al, 2006). Several studies have reported that Schizophrenia patients lack gamma band activity in prefrontal areas required for cognitive functions when compared with normal individuals, providing strong evidence for reduced GABA activity in Schizophrenia (Cho et al, 2006; Gonzalez-Burgos and Lewis, 2008). Other studies have also shown reduced GABA activity in the visual cortex leading to orientation-specific surround suppression (OSSS), a behavioral measure of visual inhibition dependent on GABAergic neurotransmission (Yoon et al., 2009; Yoon et al, 2010). Furthermore, reduced uptake (Reynolds et al, 1990) and release (Sherman et al, 1991) of GABA neurotransmitter have also been reported.
The molecules that are directly involved in GABA neurotransmission are also altered as in the other neurotransmission pathways involved in Schizophrenia. For example, several researchers in the field have provided evidence for altered GABA transporter (GAT) levels in PFC of Schizophrenic subjects (Woo et al, 1998; Volk et al, 2001). In human prefrontal cortex, the chandelier cells act as the main inhibitory GABA neurons on pyramidal cells, which are the principal type of excitatory neurons (Woo et al, 1998). In their study, Woo and his colleagues used an antibody against GAT-1 transporter in GABA neurons of postmortem brain cells of schizophrenia patients and noticed that there was a 40% reduction in GABA axon terminals with GAT-1 transporters (Woo et al, 1998). Together, these studies support the GABA hypothesis that the decreased amount of GABA neurotransmission in the affected brain areas of schizophrenia patients. Studies have also shown that decreased levels of glutamic acid decarboxylase (GAD), enzyme is important in the conversion of glutamate to GABA, in schizophrenic patients (Fatemi et al, 2005). Together, these studies explain the cognitive symptoms of schizophrenia due to decreased GABA neurotransmission.
Integration of Neurotransmitter Systems
Given the roles of each neurotransmission system in Schizophrenia, we are trying to understand how all three of these circuits would interact with each other in order to develop positive, negative, and cognitive symptoms of schizophrenia. Many studies done over the past decade addressed this question.
As mentioned earlier, the NMDA-R1 receptor knockout for glutamate leads to schizophrenia (Mohn et al, 1999). A study that was immediately followed showed that there were higher levels of dopamine in postmortem brain tissues of the striatum and the frontal cortex. These mice also displayed symptoms such as increased locomotor activity and cognitive deficits. Further, these effects could be alleviated by treatment with antipsychotics (Miyamoto et al, 2000). Recent findings also suggest that early postnatal inhibition of NMDAR activity in GABAergic inter-neurons contribute to the pathophysiology of schizophrenia (Belforte et al, 2010).
In a study done by Yee and colleagues, it was shown that GABAA receptor α3 knockout mice show positive symptoms associated with hyperdopaminergic activity (Yee et al, 2005). This might be due to the absence of inhibitory effects on dopamine neurons might be leading to hyperactivity.
All of these studies together suggest that hypofunction of NMDA receptors as a possible starting point that will lead to alterations in many neurotransmitter systems. Current evidence in the field suggests the crucial role of glutamate hypothesis in understanding the nature of schizophrenia. Recent studies have given us the opportunity of putting the puzzle pieces together and make a unified theory to explain molecular mechanisms underlying the disease. By combining the studies that have been done we can conclude that hypo-activity of NMDA receptor leads to less glutamate levels leading to a decrease in GABA synthesis. Altered GABA levels will result in hyperactivity of the dopamine neurotransmission. The possible interaction of the pathways that lead to schizophrenia is shown in figure 1.
Role of genetics in Schizophrenia
In this part of our review we will focus on candidate genes that are found to be involved in onset of schizophrenia.
DISC1 isoform regulation and mitochondrial interaction
The Disrupted-in-Schizophrenia 1 gene (DISC1) gene is involved in neurodevelopment, regulating neurite growth, neuronal migration, and neurogenesis (Nakata, 2009). Although found in both the hippocampus and lymphoblasts, DISC1 is found in different forms. The DISC1 differs between these locations because different isoforms are created through the splicing of exons. The isoforms in DISC1 include a missing exon3, missing exon7 and 8, and an exon3 insertion variant; each creating truncated DISC1 mRNA (Nakata et al, 2009). The production of these DISC1 isoforms is thought to be due to changes in gene processing during DISC1 splicing, forming aberrant or truncated DISC1 mRNA. In the hippocampus of schizophrenia patients, as well as during fetal development, DISC1 is more commonly found in the truncated isoforms. The truncated DISC1 in the hippocampus does not have the C-terminal region that is thought to be necessary for proper neurodevelopment. This may cause a deficit in neurodevelopment which may lead to the onset of schizophrenia in patients who have disrupted processing of DISC1. Although the effects of DISC1 are known, the mechanism by which DISC1 leads to schizophrenia has only recently begun to be understood.
Fig. 2: Model displaying genetic factors associated with schizophrenia. Function of de novo CNV, DISC1, NRG3, and DTNBP1 are outlined in the diagram. De novo CNV can cause a variety of issues causing schizophrenia; lack of DISC1 causes mitochondrial dysfunction; NRG3 isoforms do not interact with complex, causing improper development of dendrites; and reduced DTNBP1 causes a decrease in synaptic vesicle and neurotransmitter release.
DISC1 has been found to play a role in mitochondrial regulation through direct interaction with mitofilin (Fig. 2), an essential mitochondrial inner membrane protein (Gieffers et al., 1997; Park et al., 2010). Reducing the levels of functional DISC1 induces dysfunction in mitochondria, causing reduced levels of ATP, disrupted Ca2+ dynamics, and decreased monoamine oxidase-A (MAO-A) production (Park et al., 2010). As mentioned previously, the truncated DISC1 isoform is found to be increased in schizophrenia, leaving less functional DISC1 to act in the neuron. This leads to a decreased interaction between DISC1 and mitofilin in the mitochondria, resulting in deregulation of mitochondrial functions (Park et al. 2010). The mitochondria have been associated with schizophrenia through the synthesis of MAO-A, a preliminary molecule to dopamine, which, as mentioned earlier, plays a vital role in neurotransmission (Ben-Shachar, 2004). As the regulation of the mitochondria decreases in response to a decrease in DISC1-mitofilin interaction, the mitochondria produces less MAO-A resulting in less dopamine production. With less dopamine in the neuron, neurotransmission is hindered, and the risk of developing schizophrenia is increased (Park et al., 2010).
DTNBP1 regulation of neurotransmitter release
As schizophrenia has many possible causes, multiple genes have been found to increase susceptibility to the disorder. Another one of these potential genes is DTNBP1, which codes for the protein dystrobrevin-binding protein 1 or dysbindin. Dysbindin has been associated with schizophrenia through its assistance in regulating neurotransmission. In patients with schizophrenia, a
decrease in levels of dysbindin in the hippocampus and prefrontal cortex is thought to result in cognitive impairment
(Weickert et al., 2008; Chen et al. 2008) and is associated with glutamate and dopamine neurotransmission in the hippocampus (Numakawa et al. 2004; Kumamoto et al. 2006). The effects of dysbindin were studied by using sandy (sdy) mice as a model due to its interesting lack of dysbindin . Amperometric and electron microscopy studies showed that dysbindin regulates the amount of secretion, the release probability, and the kinetics of synaptic vesicles by regulating the fusion step of exocytosis (Chen et al., 2008). This study also showed that the kinetics of vesicle release ware altered in hippocampal nerves in sdy mice due to the loss of dysbindin alone (Chen et al., 2008). These results suggest that decreased levels of dysbindin could cause cognitive symptoms of schizophrenia symptoms by affecting the transmission of neurotransmitters. Other studies have shown that dysbindin is associated with negative symptoms of schizophrenia (DeRosse et al., 2006). As a result of dysbindin’s role in neurotransmission, genetic variations in DTNBP1 may decrease the level of dysbindin present at the synapses
of neurons (Fig. 2). This reduction of dysbindin may induce altered vesicle trafficking leading to impaired neurotransmission, and ultimately the negative symptoms associated with schizophrenia.
Neuregulin isoform affects symptoms in Schizophrenia
A third susceptibility gene, NRG3, encodes the protein neuregulin which has been shown to play a role in neurodevelopment in the cerebral cortex by aiding in cell migration and patterning (Balciuniene et al., 2007). NRG3 interacts with NRG1, ErbB4, and ATK1 to form a complex in the NRG-ErbB signaling pathway. NRG3, similarly to DISC1, goes through complex splicing to form many different isoforms that are developmentally regulated and pathologically increased in schizophrenia (Kao et al., 2010). This suggests that some variations in neuregulin may be associated with onset of schizophrenia, making NRG3 another susceptibility gene. Indeed, studies have shown that several isoforms of NRG3 are associated and elevated in the schizophrenic brain (Carteron et al, 2006). Although previously discovered genes mostly affected one classification of symptoms, NRG3 isoforms can affect positive, negative, or cognitive symptoms (Chen et al., 2009; Kao et al., 2010). Increased expression of NRG3 can lead to deficient neurodevelopment and possibly less neurotransmission (Fig. 2).
Rare de novo CNV’s associated with schizophrenia
Schizophrenia can exist in familial form, in which genetic mutations are passed from parent to offspring, and sporadic form, in which the mutations occur independently of the parents’ genetic makeup. In schizophrenia patients, many small, de novo copy number variants (CNV) create mircodeletions and microduplications. These mutations in the genome can affect genes that are associated with the disease, increasing the susceptibility of developing schizophrenia. In recent studies, CNV’s were found to be most common in early onset schizophrenia, with about 20% of early onset patients having de novo CNV mutations. Non-early onset schizophrenia also showed a significantly higher percent of cases with CNV’s than the control cases. CNV were present in about 15% of non-early onset schizophrenia while only about 5% of controls had CNV’s (Walsh et al., 2008). Also, CNV’s were found to be more common in sporadic than in familial schizophrenia (Xu et al., 2008). These studies suggest that schizophrenia can be caused by rare mutations affecting neurodevelopment and regulation pathways in the brain, such as the glutamate receptor and neuregulin signaling. As neurodevelopment contains hundreds of proteins, there are many possible mutations that will alter pathways and affect neuronal development and regulation. While each of these mutations may not occur often, the large number of different possible mutations may contribute to a significant portion of the total cases of schizophrenia. Further research of de novo CNV mutations may lead to the discovery of more genes that are involved in neurodevelopment pathways, such as the NRG-ErbB pathway, and neurotransmission pathways, including the dopamine, glutamate, and GABA neurotransmitters. Many mutations have already been identified and connected to schizophrenia through the discovery of de novo CNV mutations in schizophrenia (Stefansson et al., 2008).
Future Research
Understanding the etiology of Schizophrenia is one of the challenges that the scientific community has faced. The recent convergence of neuropathological, neurochemical, and genetic studies may lead us to understand the molecular basis and causes of schizophrenia.
Possible future studies would aim at further elucidating the interactions between genetic variations, neurotransmitter pathways, and environmental factors in order to find a link between the altered neurotransmission pathways and candidate genes in hopes of gaining insight to finding the cause of the disease. Previous studies have shown that genetic predispositions may not have an effect unless environmental pressures are present. Twin studies can help to elucidate selective environmental pressures associated with schizophrenia. Other than the complex role environment plays in the development of schizophrenia, protein-protein interactions may also add complexity to the pathological mechanism. As in the NRG-ErbB signaling complex, one protein variation among any of the proteins in the complex may lead to the same detrimental effects that would be seen if another of the proteins in the complex was varied. By understanding which protein ultimately caused detrimental effects leading to disease onset, we may be able to link the source and outcome of deficient pathways. Apart from the neurotransmitter pathways that are mentioned in this article, serotonin neurotransmission has also been found to be altered and found to be hyper-activated under decreased levels of NMDA receptors (Miyamoto et al, 2001). To understand the nature of the disease, it is necessary to combine the neurotransmission systems.
While presenting many challenges to study, schizophrenia affects the most unique human characteristics of ours: these higher order skills make it difficult to create a suitable animal model. Therefore, drawing conclusions about the disease should rely on neuropharmacology, neuroimaging, and genetic studies until a model with measureable phenotypes is created.
Conclusion
After over one hundred years since it was named, the cause and biological basis of schizophrenia remains unknown. Involvement of multiple neurotransmitter systems and genes has advanced our understanding about the disease over the past decade. The current evidence suggests that schizophrenia does not have one central cause but instead, a combination of several neurotransmitter pathway alterations and disrupted genetics.
Note: Eukaryon is published by students at Lake Forest College, who are solely responsible for its content. The views expressed in Eukaryon do not necessarily reflect those of the College. Articles published within Eukaryon should not be cited in bibliographies. Material contained herein should be treated as personal communication and should be cited as such only with the consent of the author.
References
Bonifati V., Rizzu P., van Baren M. J., Schaap O., Breedveld G. J., Krieger E., et al. (2003) Mutations in the DJ-1 gene associated with autosomal recessive early-onset parkinsonism. Science 299, 256–259.
Brandis, K., Holmes, I., and England, S. (2006) α-Synuclein fission yeast model: concentration-dependent aggregation without membrane localization or toxicity. J. Mol. Neuroscie., 28(6), 179-191.
Chen L, Feany MB. Α-synuclein phosphorylation controls neuro- toxicity and inclusion formation in a Drosophilamodel of Parkinson’s disease. Nat Neurosci 2005;8:657Y63
Chiti, F., Stefani, M., Taddel, N., Ramponi, G., and Dobson, C.M. (2003) Rationalization of the effects of mutations on peptide and protein aggregation rates. Nature 424, 805-808.
Cooper, A.A., Gitler, A.D., Cashikar, A. Haynes, C.M., Hill, K.J., Bhullar, B., Liu, K., Xu, K., Strathearn, K.E., Liu, F., Cao, S., Caldwell, K.A., Marsischky, G., Kolodner, R.D., LaBaer, J., Rochet, J.C., Bonini, N.M., and Lindquist, S. (2006) Α-Synuclein Blocks ER-Golgi Traffic and Rab1 Rescues Neuron Loss in Parkinson’s Models. Science 313, 324- 328.
Dixon, C., Mathias, N., Zwieg, R.M., Davis, D.A., and Gross, D.S. (2005) Α-Synuclein targets the plasma membrane via the secretory pathway and induces toxicity in yeast. Genetics 170, 47-59.
Fujiwara, H., Hasegawa, M., Dohmae, N., Kawashima, A., Masliah, E., Goldberg, M.S., Shen, J., Takio, K., and Iwatsubo, T. (2002). α-Synuclein is phosphorylated in synucleinopathy lesions. Nat. Cell Biol. 4, 160-164.
Giasson, B.I., Murray, I. V.J., Trojanowski, J.Q., and Lee, V.M-Y. (2001) A Hydrophobic Stretch of 12 Amino Acid Residues in the Middle of α-Synuclein Is Essential for Filament Assembly. Journal of Biological Chemistry, 276, 2380-2386.
Gorbatyuk, O.S., Li, S., Sullivan, L.F., Chen, W., Kondrikova, G., Manfredsson, F.P., Mandel, R.J., and Muzyczka, N. (2008). The phosphorylation state of Ser-129 in human α-synuclein determines neurodegeneration in a rat model of Parkinson disease. Proc. Natl. Acad. Sci. U. S. A. 105, 763-768.
Kitada T., Asakawa S., Hattori N., Matsumine H., Yamamura, Y., Yocochi M., et al. (1998) Mutations in the parkin gene cause autosomal recessive juvenile parkinsonism. Nature 392, 605–606.
Lee, V.M.-Y. and Trojanowksi, J. Q. (2006) Mechanisms of Parkinson’s Disease Linked to Pathological α-Synuclein: New Targets for Drug Discovery. Neuron 52, 33-38.
Lozano, A.M. and Kalia, S.K. (2005) New Movements in Parkinson’s. Scientific American. pp 68- 75.
McFarland, N.R., Fan, Z., Xu, K., Schwarzschild, M.A., Feany, M.B., Hyman, B.T., and McLean, P.J. (2009). Alpha-synuclein S129 phosphorylation mutants do not alter nigrostriatal toxicity in a rat model of Parkinson disease. J. Neuropathol. Exp. Neurol. 68, 515-524.
Outeiro, T.F. and Lindquist, S. (2003). Yeast Cells Provide Insight into Α-Synuclein Biology and Pathobiology. Science 302, 1772- 1775.
Paisan-Ruiz C., Jain S., Evans E. W., Gilks W. P., Simon J., van der Brug M., et al. (2004) Cloning of the gene containing mutations that cause PARK8-linked Parkinson’s disease. Neuron 44, 595–600.
Paleologou, K.E., Schmid, A.W., Rospigliosi, C.C., Kim, H.Y., Lamberto, G.R., Fredenburg, R.A., Lansbury, P.T.,Jr, Fernandez, C.O., Eliezer, D., Zweckstetter, M., and Lashuel, H.A. (2008). Phosphorylation at Ser-129 but not the phosphomimics S129E/D inhibits the fibrillation of α-synuclein. J. Biol. Chem. 283, 16895-16905.
Periquet, M. Fulga, T., Myllykangas, L., Schlossmacher, M.G., and Feany, M.B. (2007). Aggregated α-Synuclein Mediates Dopaminergic Neurotoxicity in Vivo. The Journal of Neuroscience 27(12):3338-3346.
Polymeropoulous, M. Lavendan, C., Leroy, E., Ide, S., Dehejia, A. Dutra, A. Pike, B. Root, H., Rubenstein, J., Boyer, R., Stenroos, E.S., Chandrasekharappa, S., Athanassiadou, A., Papapetropoulous, T., Johnson, W.G., Lazzarini, A.M., Duvoisin, R.C., Di lorio, G., Golbe, L.I., and Nussbaum, R.L. (1997). Mutation in the Α-synuclein Gene Identified in Families with Parkinson’s Disease. Science. 276: 2045.
Rochet, J.C., Outeiro, T. F., Conway, K.A., Ding, T.T., Volles, M.J., Lashuel, H. A., Bieganski, R.M. Lindquist, S.L., Lansubry, P.T. (2004) Interactions Among Α-Synuclein, Dopamine, and Biomembranes. Journal of Molecular Neuroscience. 23, 23-32.
Sharma, N., Brandis, K. A., Herrera, S.K., Johnson, B. E., Vaidya, T., Shrestha, R., and DebBurman, S.K. (2006). Α-Synuclein Budding Yeast Model: Toxicity Enhanced by Impaired Proteasome and Oxidative Stress. Journal of Molecular Neuroscience 28, 1559-1166.
Silveira, S.A., Schneider, R.T., Diaz, C.C., Sage, D., Terki, T.A., Iwatsubo, T., Unser, M., Aebiischer, P. (2008). Phosphorylation does not prompt, nor prevent, the formation of α-synuclein toxic species in a rat model of Parkinson’s disease. Human Molecular Genetics. 18, 872-887.
Smith, W.W., Margolis, R.L., Li, X., Troncoso, J.C., Lee, M.K., Dawson, V.L., Dawson, T.M., Iwatsubo, T., and Ross, C.A. (2005). Α-synuclein phosphorylation enhances eosinophilic cytoplasmic inclusion formation in SH-SY5Y cells. J. Neurosci. 25, 5544-5552.
Soper, J.H., Roy, S., Stieber, A., Lee, E., Wilson, R.B., Trojanowski, J.Q., Burd, C.G., and Lee, V.M. (2008). Α-synuclein-induced aggregation of cytoplasmic vesicles in Saccharomyces cerevisiae. Mol. Biol. Cell 19, 1093-1103.
Spillantini, M.G., Crowther, R.A., Jakes, R., Hasegawa, M., Goedert, M. (1998). Α-Synuclein in filamentous inclusions of Lewy bodies from Parkinson’s disease and dementia with Lewy bodies. Proc. Natl Acad. Sci. 95, 6469-6473.
Valente E. M., Abou-Sleiman P. M., Caputo V., Muqit M. M., Harvey K., Gispert S., et al. (2004) Hereditary early onset Parkinson’s disease caused by mutations in PINK1. Science 304, 1158–1160.
Yamada, M., Iwatsubo, T., Mizuno, Y., and Mochizuki, H. (2004). Overexpression of α-synuclein in rat substantia nigra results in loss of dopaminergic neurons, Phosphorylation of a-synuclein and activation of caspase-9: resemblance to pathogenetic changes in Parkinson’s disease. Journal of Neurochemistry, 2004, 91, 451-461.
Zabrocki, P., Pellens, K., Vanhelmont, T., Vandebroek, T., Griffioen, G., Wera, S., et al. (2005) Characterization of α-synuclein aggregation and synergistic toxicity of protein tau in yeast. FEBS J. 272, 1386-1400.
Zimprich A., Biskup S., Leitner P., Lichtner P., Farrer M., Lincoln S., et al. (2004) Mutations in LRRK2 cause autosomal- dominant parkinsonism with pleomorphic pathology. Neuron 44, 601–607.