Creation of α-Synuclein C-terminus Truncations at Amino Acids 110, 100, 90, & 80, for the Use in Future Research Relating to Parkinson’s Disease in Budding Yeast
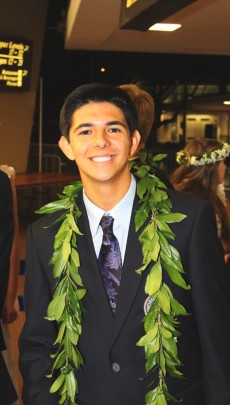
Cade Brittain, Rebecca Shoup, and Anthony Zimmerman
Department of Biology
Lake Forest College
Lake Forest, Illinois 60045
Abstract
A type of neurodegenerative disease called Parkinson’s disease is incurable and often fatal with one of its characteristic symptoms being the development of motor dysfunctions that are due to, in part, the death of dopaminergic neurons in the substantia nigra and the aggregation of Lewy bodies in this same region of the mid-brain. Alpha-synuclein is a protein, made from the SNCA gene, that has the ability to aggregate and form these Lewy bodies within the brain that are seen in Parkinson’s disease. While this linkage to Parkinson’s disease is well known, the specific role of various parts of the alpha-synuclein protein that play a role in aggregation are not. This project’s aim was to create four truncations at the 110, 100, 90 and 80 amino acids in the C-terminus region of the SNCA gene, in order to investigate the differing properties that each truncated version would have on the folding of the protein in future experiments. Our hypothesis was that each truncation would demonstrate less solubility and more aggregation as more amino acids were removed from the C-terminal region. In this project we were able to successfully transform truncations 100, 90 and 80 into E.coli and then, after PCR was used to ensure proper orientation, were able to successfully transform them into the yeast strain S.cerevisiae as well. However, we were unable to successfully identify any truncation 110 gene fragments that were correctly orientated within our vector and therefore this truncation could not be transformed into yeast. With the truncations that were successfully created, future research will be able to be done to investigate how they affected alpha-synuclein’s function and this understanding will increase our knowledge on alpha-synuclein and its relation to Parkinson’s Disease.
Introduction
Parkinson’s disease (PD) is an incurable, and often fatal, neurodegenerative disease where the death of dopaminergic neurons in the substantia nigra pars compacta leads to the expression of motor dysfunctions such as rigidity, bradykinesia, resting tremor, postural instability, flexed posture, and freezing of gait. (Keener and Bordelon 2016). While there are a multitude of secondary Parkinsonism disorders, there are only two main types of primary parkinsonism disorders: sporadic and familial idiopathic (Dauer and Przedborski 2003). While the main causes of sporadic PD are less understood, in familial idiopathic PD the causes are derived from known genetic etiology. (Nass and Przedborski 2008) Some of the many genes that have been associated with causing the Familial Idiopathic form of Parkinson’s include PINK1, UCH-L1,parkin,DJ-1, LLKR, and SNCA (de Lau and Breteler 2006).
SNCA in particular, is a gene of interest in relation to PD due to it being the major component in the aggregation of Lewy bodies, which are clumps of misfolded α-synuclein protein, in the mid-brain which is one of the most recognizable traits of Parkinson’s (Breydo et al. 2012). In addition to this, while the exact function of α-synuclein remains relatively unknown, suggestions of possible functions include involvement in synaptic vesicle release and trafficking, fatty acid binding and physiological regulation of certain enzymes, transporters, and neurotransmitter vesicles, as well as some roles in neuronal survival (Dev et al. 2003). Regardless of the exact function of α-synuclein, its capacity to aggregate and the factors that affect its conformation are strongly related to the biological bias of PD. (Breydo, et al. 2012). Although some of these functions have been demonstrated, there is still a gap of knowledge pertaining to which parts of the α-synuclein protein are responsible for its aggregation.
Areas that are known to contain high concentrations of α-synuclein include both soluble and membrane-associated fractions of the brain and presynaptic terminals. (Iwai et al. 1996) In these locations, α-synuclein is a prominent brain protein that consists of a sequence of 140 amino acids. This sequence is able to be divided into three separate domains: amino acids 1-60 represent the N-terminal domain, 61-95 represents the M-domain and 96-140 represents the C-terminal domain. It is hypothesized that each of these domains performs a specific function associated with its region. The N-terminal domain and the M-domain are both involved with membrane-binding for α-synuclein with amino acids K96 and S87 being located in the M-domain specifically. In contrast the C-terminal domain is believed to be specified for protein-protein and protein-small molecule interaction sites (Breydo et al. 2012) and contains the amino acids Y136, Y133, S129, Y125, K102 and K96
The three most important types of modifications that occur in α-synuclein are SUMOylation, nitration and phosphorylation. SUMOylation is a modification that adds small ubiquitin-like modifier (SUMO) protein groups to lysine in proteins (Hendriks and Vertegaal, 2016) which provides a protective effect to the protein as it decreases α-synuclein’s ability to aggregate and increases its solubility (Krumova et al. 2011). This particular protective effect occurs at amino acids K96 and at K102 in SNCA with the effect being greater in K102 than when it occurs on its counterpart K96 (Abeywardana and Pratt 2015). Nitration is a modification where a nitro group, most likely being nitric oxide, is added to tyrosine in proteins (Uversky and Lyubchenko, 2013). This modification occurs at amino acids Y125, Y133 and Y136 of SNCA and decreases the ability of α-synuclein to bind with membranes (Sevcsik et al. 2011). In addition to amino acid Y133’s membrane binding influences, it has been implicated to have a protective function as well to the phosphorylation of S129 (Kleinknecht et al. 2016). Phosphorylation is a modification where a phosphate group is added to serine in proteins so therefore this modification occurs amino acids S129 and S87 in SNCA. In S87 phosphorylation provides additional protection against aggregations well as a decrease of the mortality rate of dopamine neurons in the brain tissue of rats (Oueslati et al. 2012). It has also been found that blocking the phosphorylation that usually occurs at S129 increases the toxicity within the cell and blocks an autophagy pathway, which in turn leads to increased aggregation (Tenreiro et al. 2014). In relation to this, the phosphorylation of Y125 is also required for the proper modification of amino acid S129 (Kosten 2014). Consequently, the modifications that occur on the amino acids in the M-domain and C-terminal domain, in particular to this study, are important when considering the molecular interaction of α-synuclein in relation to PD.
In this experiment, truncations in entirely in the C-terminal domain and partly in the M-domain will be made after amino acids 110, 100, 90 and 80. Beginning with truncation 1-110, the amino acids Y135, Y133, Y125, and S129 will be cut from the gene which then may affect the lipid binding and cell autophagy properties of the SNCA gene. As a result, it is expected that after the 1-110 truncation, a decrease in α-synuclein’s ability to properly bind with membrane, and an increased likelihood of aggregation will be observed. Next in truncation 1-100, amino acid K102 will be lost, which is vital in maintaining α-synuclein’s ability to be soluble. Thus, it would be expected that after the 1-100 truncation, the ability of α-synuclein to be soluble in water would have an observable decrease. For truncation 1-90 there will be the loss of K96, and while it is not as important in solubility as K102, it can be expected that an even greater decrease in α-synuclein soluble ability will be observed. Lastly in truncation 1-80, there will be an elimination of the amino acid S87, which when phosphorylated has a protective ability against aggregation, therefore after truncation it can be expected to observe an increase in α-synuclein aggregation.
The objective of this project, was to successfully create the four truncated versions of the SNCA gene (1-80, 1-90, 1-100, and 1-110) to allow for the future study of the different properties that each truncation demonstrated. These truncations were created using primers and PCR amplification. The truncated genes were then subcloned into a vector. The vector was transformed into the bacteria and grown on an ampicillin petri dish, and then checked via PCR and gel electrophoresis to assure that the gene had been subcloned in the right orientation. The vector was then transformed into yeast on SC-Ura plates.
For this project Saccharomyces cerevisiae, commonly known as budding yeast, will be used as the model organism. It has been found to be one of the most effective organisms in studying human genes, as well as the most studied eukaryotic cell, because it provides the greatest insight in studying protein mis-folding which is particularly useful for neurodegenerative diseases specifically caused by protein mis-folding such as Parkinson’s. This is due to yeast having one of the smallest genomes of any free living organism, which makes them easy to study due to the high speed at which the replication and synthesis of proteins occurs, and also because a majority of its sequence motifs are shared with human proteins which therefore makes it possible to swap yeast genes for human counterpart genes with the overall functionality remaining intact (Allendoerfer et al., 2008). Yeast are also able to make, fold and degrade proteins similar to the way we do which allows their results in experiments to be applicable to what happens in humans. In particular, the gene SNCA has been studied extensively using yeast models mainly with how its heterologous expression in yeast inhibits growth and promotes cell death (Outeiro and Lindquist, 2003). Other major elements of PD in addition to this, that have also been accurately demonstrated in budding yeast, are those such as the formation of intracellular foci (Outeiro & Lindquist, 2003; Dixon et al., 2005; Zabrocki et al., 2005; Gitler et al., 2008) and oxidative stress (Flower et a.2005). Some other model organisms that are useful for studying genetics in eukaryotes are fruit flies, worms and zebrafish as they easily show how mutations in genes affect the physical development and growth of an organism. However, in relation to certain protein abilities such as aggregation levels and having a quick replication speed, they are not as useful as yeast.
Results
Overall Project Design and Gene Fragment Amplification by PCR
The aim of the project was to create four unique truncated fragments 1-110,1-100,1-90, and 1-80 of the SNCA gene that makes the protein alpha-synuclein by removing amino acids from the C-terminus end (Figure 1A). In order to create these gene fragments, four unique reverse primers and one common forward sequence were designed and ordered prior to the amplification process by Emily Ong (Figure 1B). The four reverse primers were also tested by PCR to ensure that they could successfully amplify DNA (Figure 1C). The first step after the primers were tested, was to then amplify the desired gene fragments of the GFP tagged SNCA gene over 29 cycles of PCR by combining differing volumes of SNCA DNA template, master mix, sterile RNase-free water, the common forward primer, all with each unique reverse primer (See method Plasmid-based PCR for full explanation of this process). This gene fragment amplification was the first step out of six that were carried out over a six-week period (Figure 1D). The other five steps illustrated in Figure 1D, in chronological order are (2) gene fragment purification by gel electrophoresis, (3) subcloning into plasmid vector & bacterial transformation, (4) orientation check I: plasmid prep & plasmid based PCR, (5) orientation check II: bacterial & plasmid PCR gel electrophoresis, and (6) plasmid vector transformation into yeast.
Figure 1. Overall Project Design and Gene Fragment Amplification
(A) Entire wild type ɑ-synuclein marked with GFP compared to predicted modifications to ɑ-synuclein by C-terminus truncations at amino acids 110, 100, 90, & 80. (B) The four reverse primers and one common forward primer used during PCR amplification. (C) Schematic of overall project design by representing the six steps. (D) Visualization gel verifying purification of all C-terminus reverse primers for this lab made by Emily Ong (in lane 4: 1-110, lane 5: 1-100, lane 6: 1-90 and lane 7: 1-80).
Gene Fragment Purification by Gel Electrophoresis
Gel electrophoresis was applied to successfully purify the desired gene fragments after they were made and amplified by PCR. The expected band sizes and location from SNCA gene fragments 1-110, 1-100, 1-90, and 1-80, along with both positive and negative controls, were predicted on a computerized model gel (Figure 2A, 2B, and 2C). Purification gel #1 displayed bands for the 1-100, 1-90, and 1-80 fragments, all around the 1kb mark (Figure 2D). However, purification gel #2 did not display any bands for 1-110 fragments but did show a band for 1-80 (Figure 2E). For the controls, a band was visible for the positive control while no band was visible for the negative control. After gel electrophoresis was complete, the desired gene fragments were extracted manually from gel#1 lane 2 (1-100 fragment with 4ul template), lane 3 (1-90 fragment with 1ul template), and lane 5 (1-80 fragment with 1ul template) (Figure 2F). The positive control did not work as demonstrated by the absence of a band (Figure 2G). Because our truncation 1-110 did not display a band, the PCR product of truncation 1-110 from group 2, which had a band displayed around the 1kb mark in lane 4 was used as its replacement in the next step (Figure 2H). The GENECLEAN Turbo Kit was then utilized to further isolate gene fragments from the remaining agarose gel.
Figure 2. Gene Fragment Purification by Gel Electrophoresis
(A) Ideal-computerized gel for purification gel #1. (B) Ideal-computerized gel for purification gel #2. (C) Ideal-computerized gel for visualization gel. (D) Purification gel #1 before removal of desired bands. (E) Purification gel #2, which contained 1-110 fragment taken from group 3 in lanes 1 and 2 highlighted in red, before the removal of desired bands. (F) Purification gel #1 after removal of desired bands containing gene amplified gene fragments. (G) Visualization gel to represent success of PCR and gel electrophoresis by showing positive and negative control bands. (H) Visualization gel to represent isolation and purification of all desired fragments was successful with fragment 1-110 from group 2 used for following steps highlighted in red in lane 4.
Subcloning into Plasmid Vector & Bacterial Transformation
The purified PCR products were then transformed into plasmid vectors for expression into bacteria. The E. coli were then plated onto plates which contained ampicillin. Typically, bacteria would die in the presence of ampicillin however, because the plasmid that was used contained the gene for β-lactamose, which gives ampicillin-resistance, the bacteria that were able to take up the closed plasmid were predicted to grow.
Each transformation was plated in 40ul and 200ul amounts, along with two negative cloning plates, a negative and positive control plate and an open vector plate. For the transformation plates, both the 40ul and 200ul plates showed growth for truncations 1-100 (Figure 3A), 1-90 (Figure 3B), 1-80 (Figure 3C) and 1-110 (Figure 3D). In both negative cloning plates, no growth was seen as expected (Figure 3E). However, while the expected results occurred for the positive control plate since growth was seen, there was also growth seen on the negative control and open vector plates which was not expected (Figure 3F). Dr. Wilcox also retransformed the truncations 1-90 and 1-110 plates along with the positive and negative control plates. On his truncation plates growth was seen on the new 1-90 truncation (Figure 3G) and 1-110 truncation (Figure 3H) as expected. In addition to this, for Dr. Wilcox’s controls, the new negative control plate showed no growth as expected (Figure 3I) and the new positive control plate showed growth as expected (Figure 3I). Then Sixteen isolated colonies were chosen from the transformation plates to perform plasmid-based PCR in order to check the orientation of the gene fragments.
Figure 3. Subcloning into plasmid vector and bacterial transformation
(A-F) Original bacterial subcloning plates displaying growth or no growth of E. Coli colonies for fragments 1-100, 1-90, and 1-80 1-110 respectively. (G) Regrown bacterial subcloning plates by Dr. Wilcox for fragment 1-90. (H) Regrown bacterial subcloning plates by Dr. Wilcox for fragment 1-110.(I) Regrown controls by Dr. Wilcox to prove regrown bacterial subcloning plates were successful.
Orientation Check II: Plasmid Prep & Plasmid Based PCR Gel Electrophoresis
After transformation the orientation of the gene fragment could be inserted in one of two ways. In order to confirm that the transformation was inserted in the proper way, plasmid based PCR was conducted for each desired truncation, 1-110, 1-100, 1-90 and 1-80. First the plasmid results were predicted for each truncation 1-110, 1-100, 1-90 and 1-80 (Figure 4A and 4B). Then two gels were loaded. Gel #1 contained the purified plasmids of truncations 1-110 on top and 1-90 on bottom. No colonies of truncation 1-110 displayed a band at the expected length, while colony 5 for truncation 1-90 in lane 6 displayed one dark band at the expected length (Figure 4C). Gel #2 contained the purified plasmids of truncations 1-100 on top and 1-80 on bottom. Colony 4 for truncation 1-100 in lane 5 displayed a faint band at the expected length, while no bands were displayed at the correct length for truncation 1-80 (Figure 4D). Because we needed at least two bands for the next step we borrowed purified plasmids that displayed a band from Dr. Wilcox and product from group 1. From Dr.Wilcox we used truncation 1-100 from colony 5 in lane 6 (Figure 4E) and truncation 1-80 from colonies 5 and 7 in lanes 6 and 8 respectively (Figure 4F). Then from group 1 we used truncation 1-90 colony 8 in lane 8 (Figure 4G). The plasmid vectors that contained a positive band were then able to be transformed into yeast cells.
Figure 4. Orientation Check II: Bacterial & Plasmid PCR Gel Electrophoresis (A-B) Ideal-computerized gels of plasmid-purified PCR products for gel #1 fragments 1-110 top and 1-90 bottom and gel #2 fragments 1-100 top and 1-80 bottom respectively. (C-D)Gel photographs of plasmid-purified PCR products to confirm correct or incorrect orientations of inserted gene fragments 1-110 top and 1-90 bottom from gel #1 and 1-100 top 1-180 bottom from gel #2 respectively within TOPO-pYES2 vectors. (E-F) Gel photograph of plasmid-purified PCR products to confirm correct or incorrect orientations of inserted gene fragments 1-100 and 1-80 within TOPO-pYES2 vectors made by Dr. Wilcox with the colony bands we used highlighted in red. (G) Gel photograph of plasmid-purified PCR products to confirm correct or incorrect orientations of inserted gene fragments 1-100 within TOPO-pYES2 vectors made by group 1 with the colony band we used highlighted in red.
Plasmid Vector Transformation into Yeast
Yeast cells exposed to lithium acetate, along with PEG, water, and a single-stranded carrier DNA were mixed with the Plasmid DNA. Six different plasmids were transformed, one for each truncation (two for 1-80, two for 1-90 and two for 1-100). Truncation 1-110 was not transformed into yeast, as there was no band displayed for any of the purified plasmids of truncation 1-110. Yeast were then plated onto a plate of SC–Ura and YPD. For each version of each truncation, there was a plate where 200ul of the cells were plated onto SC-Ura, 30ul of the cells were plated onto SC-Ura and 30ul of the cells were plated onto a YPD plate. For plasmid 1-100 #4, growth was seen on all three plates (SC-Ura 200ul, SC-Ura 30ul, and YPD plate) (Figure 5A). For plasmid 1-100 Dr. Wilcox #5, growth was seen on all three plates (SC-Ura 200ul, SC-Ura 30ul, and YPD plate) (Figure 5B). For plasmid 1-90 group one #8, growth was seen on all three plates (SC-Ura 200ul, SC-Ura 30ul, and YPD plate) (Figure 5C). For plasmid 1-90 #5, growth was seen on all three plates (SC-Ura 200ul, SC-Ura 30ul, and YPD plate) (Figure 5D). For plasmid 1-80 Dr. Wilcox #7, growth was seen on all three plates (SC-Ura 200ul, SC-Ura 30ul, and YPD plate) (Figure 5E). For plasmid 1-80 Dr. Wilcox #5, growth was seen on all three plates (SC-Ura 200ul, SC-Ura 30ul, and YPD plate) (Figure 5F). For the positive control on the SC-Ura plate growth was seen, while for the negative control on SC-Ura plate, some growth (a few small colonies) was seen (Figure 5G). Then for both the positive and negative control on YPD plate growth was seen (Figure 5H).
Figure 5. Plasmid Vector Transformation into Yeast
(A-F) Pictures of yeast transformation plates displaying successful colony growth for fragments 1-100, 1-90, and 1-80 respectively with 1-100 #5, 1-80 # 5, 1-80 #7 from Dr. Wilcox. (G) Pictures of positive and negative control yeast transformation on SC-Ura plates. (H) Pictures of positive and negative control yeast transformation on YPD plates.
DNA Sequencing
The results of the DNA sequencing of truncations 1-100, 1-90 and 1-80 from the University of Chicago came back as inconclusive (1-110 was not sent for sequencing).
Discussion
The overall aim of the project was to successfully create four SNCA fragments by performing C-terminal truncations at amino acids 110, 100, 90 and 80, which will be significant in aiding future Parkinson’s disease research related to the investigation of the specific properties of certain regions of the SNCA gene. Referring to the introduction, it was hypothesized that by removing the entire C-domain, part of the M-domain, and critical amino acids, there will be property alterations in the ɑ-synuclein protein product as a result. For example, in brief terms, the 1-110 fragment will have an increased likelihood of aggregation, the 1-100 fragment will have altered solubility properties, the 1-90 fragment will have an even greater decrease in solubility, and lastly the 1-80 fragment will also be expected to observe an increase in α-synuclein aggregation. The general findings that resulted from this project was the creation of the 3 C-terminal truncations 1-100, 1-90 and 1-80 that were successfully subcloned into yeast in the correct orientation which was verified by a PCR check however, their sequences are not yet verified as the DNA sequencing results were inconclusive. In addition to this, the C-terminal truncation 1-110 was not successfully made even though it was successfully amplified by PCR, purified by gel electrophoresis and subcloned into bacteria, as it had no colonies that were successfully sublconed into yeast in the correct orientation and therefore was overall not successful and so its DNA sequence was not verified.
In the first step (Gene Fragment Amplification by PCR) and in the second step (Gene Fragment Purification by Gel Electrophoresis), we were successfully able to both amplify and purify the desired gene fragments,1-100, 1-90, and 1-80 but not fragment 1-110. Gene fragment amplification of the successful fragments was confirmed by gel electrophoresis because positive bands were displayed at correctly predicted base pairs on our purified gel #1 r(Figure 2D). However, as mentioned previously, gene amplification and purification for fragment 1-110 was not successful as no bands were displayed at the predicted base pairs on purified gel #2 where the PCR product was received from a no longer existing Group 3 (Figure 2E) We know the displayed gene fragment bands to be valid because we had a band displayed for the positive control lane and no band displayed for the negative control lane on our gel (Figure 2G). Due to group 3’s 1-110 fragment not working we borrowed the successfully amplified and purified 1-110 fragment from group 2 seen as there was a successfully displayed band at the correct base pair (Figure 2H). The conformations by gel electrophoresis indicated that we were ready to separate and isolate successfully truncated gene fragments from the agarose gel.
After isolating the three successful gene fragments, 1-100, 1-90, and 1-80, (and obtaining group 2’s 1-110) we were ready for the third step (Subcloning into Plasmid Vector & Bacterial Transformation). We then proceeded to insert the four gene fragments into budding yeast TOPO-pYES2 vectors, which were then transformed into E. Coli bacterial cells. E. Coli bacterial cells with successful transformations will possess closed circular vectors containing an ampicillin resistance gene. Typically, LB+AMP plates would eliminate all bacteria. However, bacterial cells containing the ampicillin resistance gene will demonstrate resistance to ampicillin and therefore be capable of multiplying and surviving. We were able to see bacterial colony growth on all expected growth plates (Figures 3A-D). Unfortunately, bacterial cell growth had also occurred on negative controls which implicated contamination (Figure 3F). It was hypothesized that the LB+AMP plates used were outdated and ineffective. In response, Emily Ong made new LB+AMP plates which then Dr.Wilcox retransformed all original PCR products onto, to verify successful cloning of the original truncations. While our 1-100 and 1-80 regrew our 1-90 and 1-110 did not which implicates they didn’t successfully clone into bacteria. So Beth Herbert re-plated our original 1-90 and 1-110 fragments to be transformed into bacteria on the new LB+AMP plates again using a quadrant streak method to ensure proper bacterial growth. Which were then retransformed by Dr. Wilcox successfully along with successful controls to verify the results as there was no growth for the negatives and growth for the positive as expected (Figure 3G-F). From these new successful bacterial plates, Emily chose, labeled and transferred 8 bacterial colonies to liquid LB+AMP in preparation of confirming gene fragment orientation in the bacteria vectors.
The fourth step (Orientation Check I: Plasmid Prep & Plasmid Based PCR) and fifth step (Orientation Check II: Bacterial & Plasmid PCR Gel Electrophoresis) were carried out to confirm correct gene fragment orientations within the vectors in the E. Coli bacterial cells. It is possible for gene fragments to have been inserted into TOPO-pYES2 vectors with either the correct or incorrect orientation. In the correct orientation, the gene fragment must be aligned with the gene promoter that is on the immediate 5’ end of the subcloning site of the vector. Any of the 8 selected colonies for each of the four fragments could have a 50% chance to be either orientation. To test orientation, we purified the plasmid from E. Coli using an alkaline treatment and conducted PCR on the purified plasmid using a forward primer that binds the gene promoter and a reverse primer that binds the 3’ end of the gene fragments. Unfortunately, only colony #5 for the 1-90 fragment and colony #4 for the 1-100 fragment displayed a band, indicating a correct orientation (Figure 4C-D). Fragments 1-110, 1-100, and 1-80 did not display and bands, indicating that orientations were incorrect (Figure 4C-D). The majority of the classes fragments presented incorrect orientations. Therefore, Dr. Wilcox used PCR products from step two and repeated steps three through five. Dr. Wilcox was able to produce several colonies where bands were displayed that showed correct orientations for fragments 1-80 and 1-100 (Figure 4E-F) and we also used a correct orientation from group 1 as positive bands were displayed for fragment 1-90 (Figure 4G) which were to be used in the last step of transforming the plasmid vector into yeast.
The final step, (Plasmid Vector Transformation into Yeast) is a method of inserting the TOPO-pYES2 vector into the BY4741 yeast strain. Yeast possess a cell wall making insertion of plasmids more difficult. The techniques used were electroporation by using electricity while including LiAc, and spheroplasting, which is a method that indices digestion of the yeasts cell wall by an enzyme. The BY4741 yeast strain had been previously modified to have its Ura3 gene knocked out. The TOPO-pYES2 vectors inserted into the yeast DNA include Ura3 gene. Therefore, without successful vector transformation, which contains the Ura3 gene, the yeast would be uracil deficient and unable to survive and multiply on an SC-Ura plate. Colony growth was observed in all plates that were expected to contain yeast growth (Figure 5A-F). The controls also showed expected growth or no growth which verified the results (Figure 5G-H). As on the SC-URa nothing should grow if it doesn’t have the correct properties to survive and on YPD anything will grow even if it doesn’t have the correct properties which is why there is growth on the negative control that is not considered contamination.
Since the correctly orientated gene fragments 1-100, 1-90 and 1-80 came back as inconclusive for their DNA sequences, we cannot say for certain if they are verified. Therefore, more samples will need to be sent to the University of Chicago in order to make sure they are verified before they can be used in future experiments.and 1-80, (and obtaining group 2’s 1-110) we were ready for the third step (Subcloning into Plasmid Vector & Bacterial Transformation). We then proceeded to insert the four gene fragments into budding yeast TOPO-pYES2 vectors, which were then transformed into E. Coli bacterial cells. E. Coli bacterial cells with successful transformations will possess closed circular vectors containing an ampicillin resistance gene. Typically, LB+AMP plates would eliminate all bacteria. However, bacterial cells containing the ampicillin resistance gene will demonstrate resistance to ampicillin and therefore be capable of multiplying and surviving. We were able to see bacterial colony growth on all expected growth plates (Figures 3A-D). Unfortunately, bacterial cell growth had also occurred on negative controls which implicated contamination (Figure 3F). It was hypothesized that the LB+AMP plates used were outdated and ineffective. In response, Emily Ong made new LB+AMP plates which then Dr.Wilcox retransformed all original PCR products onto, to verify successful cloning of the original truncations. While our 1-100 and 1-80 regrew our 1-90 and 1-110 did not which implicates they didn’t successfully clone into bacteria. So Beth Herbert re-plated our original 1-90 and 1-110 fragments to be transformed into bacteria on the new LB+AMP plates again using a quadrant streak method to ensure proper bacterial growth. Which were then retransformed by Dr. Wilcox successfully along with successful controls to verify the results as there was no growth for the negatives and growth for the positive as expected (Figure 3G-F). From these new successful bacterial plates, Emily chose, labeled and transferred 8 bacterial colonies to liquid LB+AMP in preparation of confirming gene fragment orientation in the bacteria vectors.
The fourth step (Orientation Check I: Plasmid Prep & Plasmid Based PCR) and fifth step (Orientation Check II: Bacterial & Plasmid PCR Gel Electrophoresis) were carried out to confirm correct gene fragment orientations within the vectors in the E. Coli bacterial cells. It is possible for gene fragments to have been inserted into TOPO-pYES2 vectors with either the correct or incorrect orientation. In the correct orientation, the gene fragment must be aligned with the gene promoter that is on the immediate 5’ end of the subcloning site of the vector. Any of the 8 selected colonies for each of the four fragments could have a 50% chance to be either orientation. To test orientation, we purified the plasmid from E. Coli using an alkaline treatment and conducted PCR on the purified plasmid using a forward primer that binds the gene promoter and a reverse primer that binds the 3’ end of the gene fragments. Unfortunately, only colony #5 for the 1-90 fragment and colony #4 for the 1-100 fragment displayed a band, indicating a correct orientation (Figure 4C-D). Fragments 1-110, 1-100, and 1-80 did not display and bands, indicating that orientations were incorrect (Figure 4C-D). The majority of the classes fragments presented incorrect orientations. Therefore, Dr. Wilcox used PCR products from step two and repeated steps three through five. Dr. Wilcox was able to produce several colonies where bands were displayed that showed correct orientations for fragments 1-80 and 1-100 (Figure 4E-F) and we also used a correct orientation from group 1 as positive bands were displayed for fragment 1-90 (Figure 4G) which were to be used in the last step of transforming the plasmid vector into yeast.
The final step, (Plasmid Vector Transformation into Yeast) is a method of inserting the TOPO-pYES2 vector into the BY4741 yeast strain. Yeast possess a cell wall making insertion of plasmids more difficult. The techniques used were electroporation by using electricity while including LiAc, and spheroplasting, which is a method that indices digestion of the yeasts cell wall by an enzyme. The BY4741 yeast strain had been previously modified to have its Ura3 gene knocked out. The TOPO-pYES2 vectors inserted into the yeast DNA include Ura3 gene. Therefore, without successful vector transformation, which contains the Ura3 gene, the yeast would be uracil deficient and unable to survive and multiply on an SC-Ura plate. Colony growth was observed in all plates that were expected to contain yeast growth (Figure 5A-F). The controls also showed expected growth or no growth which verified the results (Figure 5G-H). As on the SC-URa nothing should grow if it doesn’t have the correct properties to survive and on YPD anything will grow even if it doesn’t have the correct properties which is why there is growth on the negative control that is not considered contamination.
Since the correctly orientated gene fragments 1-100, 1-90 and 1-80 came back as inconclusive for their DNA sequences, we cannot say for certain if they are verified. Therefore, more samples will need to be sent to the University of Chicago in order to make sure they are verified before they can be used in future experiments.
Future Experiments
In the future, the resulting three successful C-terminus truncations from this project, can be further analyzed as to how they will affect the functionality of alpha-synclein in the S.cerevisiae yeast the strain. It was hypothesized that due to the loss of specific amino acids in each truncation of the SNCA gene, that the aggregation levels of alpha-synuclein will increase and plasma membrane solubility will decrease. Therefore future experiments can be done to test whether this hypothesis is supported.
In order to examine the effects on aggregation and solubility from the gene truncations, it will be important to first visualize where the expression of the truncated SNCA genes are in the S.cerevisiae to know where to test for these changed properties. For this process fluorescence microscopy can be used as it is a useful method in being able to show the expression of genes in living cells and organelles. The truncated SNCA genes from this project were tagged with GFP which stands for, green fluorescent protein marker. Previous studies have shown that when cells contain genes that are tagged with this marker, wherever the gene is expressed will glow green. (Rizzuto, R. et al. 1995) Therefore the location of where the truncated genes are expressed within the yeast will be able to be seen. After that step other methods will be able to be used to examine the any change of aggregation and solubility properties. For example, to detect SUMO levels and if their interactions with SNCA have been modified leading to difference in solubility and aggregation effects, proximity ligation assay(PLA) technology can be used. This assay allows for easy visualization of the protein-protein interactions as well as being able to detect the location of these protein modifications. It works by using antibodies and complementary oligonucleotides which are amplified with a fluorescent probe that binds to the SUMO locations with each spot highlighting a protein-protein interaction (Ristic et al., 2016). In addition to this, different aggregation patterns that may be present in different fragments could be visualized by using electron microscopy. An electron microscope would bounce electrons off of the aggregates structure providing it’s shape and size.(Bungeroth et al., 2014)
In conclusion three successful C-terminal truncations 1-100, 1-90 and 1-80 were created in the correct orientation in yeast while the 1-110 truncation was not produced in the correct orientation in yeast. Due to the DNA sequencing results being inconclusive for the 3 truncations, first new samples will need to be sent to the University of Chicago to verify their sequences as correct before they can be used in future experiments. The significance of these findings is that the 3 successful truncations of the SNCA gene made can now be used to further investigate their specific effects on the properties of certain α-synuclein regions in relation to its solubility and aggregation properties in Parkinson’s disease by using assays such as PLA, electron microscopy and fluorescence microscopy.
Material & Methods
Primer Design and Synthesis
First, the primers of the specific truncations that were used in this project were designed and then made in accordance to the (DebBurman, Wilcox 2016) lab manual. The four distinct reverse primers and the common forward primer paired with each reverse primer were designed by referencing the known 140 amino acid sequence of α-synuclein. For the common forward primer among all truncations, the first 39 nucleotides were used reading from a 5’ to 3’ direction on the coding strand. For the four distinct reverse primers the C-terminus was truncated at the 110, 100, 90 and 80 nucleotides. So the reverse primers contained 39 nucleotides as well but instead of starting from the beginning of the sequence on the coding strand, started from their desired truncated region, was read going in the 5’ to the 3’ direction on the template strand (See Figure 2B for sequences).
Template DNA, Subcloning Plasmids, and Bacterial and Yeast Cells Used
The template for DNA that was used in this project was taken from the SNCA gene, which codes for the α-synuclein protein, that was then put into a plasmid vector (DebBurman, Wilcox 2016). The specific plasmid vectors that were used in the process of sublconing the desired gene fragments from this gene were PYES2.1. The reason this type of plasmid vector was chosen was due to it containing the gene Ura3. This particular gene is important as it is ampicillin resistant and produces the nucleic acid uracil which is needed for normal protein expression to occur. Both of these functions are essential in order for the bacteria E.coli to survive on SC-Ura plates. This is because uracil is not present on them, so therefore there needs to be a source of uracil for the E.coli to use in order for it to express the desired gene SNCA, and the ampicillin present on them would normally kill the E.coli as well so it needs to gain that resistance to survive and grow. Along with E.coli, these plasmid vectors were also able to be used with the S.cerevisiae yeast strain as well as it ,again, provides the source of uracil in order for the S.cervisiae to express the desired gene SNCA. For yeast this the ampicillin resistance is a less important factor.
Plasmid-based PCR
Polymerase chain reaction (PCR) functions by using an alternating thermal cycle that leads to the amplification (an increase in amount) of desired the gene fragments (1-110,1-100,1-90,1-80) from the initial plasmid vectors. To amplify the specific gene fragments, PCR uses a combination of primers and DNA polymerase that through temperature changes are continuously activated so that, by the end of the repeated thermal cycle, there are many copies of the gene fragment present, which then makes the isolation of that DNA fragment more accessible. Before this process was done using the desired gene fragments, it first needed to be tested with a positive and negative control to ensure it was working properly and that the results of the PCR would not be affected by any malfunction in this process. First a positive control was run using the primer GFP-SNCA 1-90 that was previously verified by peer teacher Emily Ong to work. Along with this verified primer the other essential components of sterile RNase-free water, Master Mix, a Forward Primer, Reverse Primer and a plasmid where also combined to create the positive control. Then two negative controls were run, where one contained no plasmid and the other contained no primer but every other component was included. These two negative controls show how both the plasmid and primer along with the other components all have to be present in order for the PCR to work.
After these controls where run and proved that the PCR was working properly, then the desired gene fragments could be amplified using PCR. There are three main temperature changes that occur in one entire cycle of PCR and the cycle was repeated a total of twenty-nine times before being stored. The first temperature was 95°C for a total of thirty seconds in order to cause denaturation to occur and unwind the DNA double helix to make the strands more accessible to the primers and DNA polymerase. Then the temperature was decreased to 55°C for thirty seconds to allow the primers to bind to the now single stranded DNA strands that serve as the templates. Then the temperature was raised to 72°C for thirty seconds to allow DNA polymerase to be activated and synthesize the complimentary strands from the template strands. After this whole process occurs twenty-nine time Then the temperature remains at 72° for thirty minutes to make sure all the strands where copied completely before being stored at 4°C.
PCR Purification
After the PCR products were made, they were purified by using the GENECLEAN Turbo Kit to perform gel electrophoresis to separate the desired amplified gene fragments by size from the other unnecessary components leftover from PCR, which includes any incomplete gene fragments, DNA polymerase and primers. The components were separated by running a charge through the gel with a negative charge on one end and a positive charge on the other, which causes the DNA to move towards the positively charged end. Depending on the predicted size of the fragment, a 2kb or 10kb ladder was used to help identify the desired gene fragment bands from the unnecessary components for each truncation after the separation. After the purified gene fragments where cut out of the gel with the aid of a UV light source to visualize the bands, they were stored at -20°C until they were used in the vector subcloning for the transformation into E.coli. The procedure for creating the gel and loading the PCR product was done according to the (DebBurman, Wilcox 2016) lab manual.
Vector Subcloning and Bacterial Transformation
After the PCR product was purified so that only the specific truncated gene fragments remained, they were then transformed into E.coli through the use of a TOPO pYES 2.1 vector subcloning process. This process allows for only the bacteria that obtained the plasmid containing the gene fragments in the correct orientation to grow, since these plasmids also were the ones that contained the ampicillin resistance and uracil production needed for the bacteria to survive and therefore these colonies can then be transferred into S.cerevisiae yeast. E. coli are used as the first transformation because of their ability to replicate quickly and their lack of a cell wall which allows for easier transformation than their counterpart S.cervisiae.
DNA Sequencing
After purification of the vectors, if a band appeared that would implicate a correct orientation so therefore that vector would be sent to the University of Chicago to be sequenced. However, if no band appeared than that meant it was subcloned in the wrong orientation and therefore would not be sent for sequencing.
Yeast Transformation
The strain of yeast that was used (BY4741) do not have the innate ability to create uracil, which is essential in the process to create RNA and thus proteins. The plasmid vector pYES2.1 TOPO-TA contains a URA3 gene, which allows the yeast to create uracil innately. The mixture that was put in with the yeast to allow transformation to occur included lithium acetate, denatured salmon sperm DNA, pYES2.1 TA Vector, PEG-3350, and sterile water. This mixture was then plated onto SC-Ura petri dishes, and YPD dishes. SC-Ura petri dishes used 200ul of the yeast mixture and 30ul of the yeast mixture. The negative and positive controls were conducted on YPD plates, as YPD contains uracil, and thus to grow, yeast do not need to contain the gene URA3. The plates were then incubated at approximately 30° C for three to five days.
Acknowledgements
Thank you to Dr. Wilcox for teaching our section of Biology 221 Lab, and for redoing so many of our transformations. Also thank you to Dr. DebBurman for teaching Biology 221 and writing the lab course along with Dr. Wilcox. In addition, thank you so much to our peer teacher Emily Ong for getting up at 6 a.m. to grow the yeast and doing so much work outside of lab in order to keep us on track.
Note: Eukaryon is published by undergraduates at Lake Forest College, who are solely responsible for its content. The views expressed in Eukaryon do not necessarily reflect those of the College.
References
Abeywardana, T. (2015). Extent of inhibition of alpha-synuclein aggregation in vitro by SUMOylation is conjugation site- and SUMO isoform selective. Biochemistry 54, 959-961.
Baba, M., Nakajo, S., Tu, PH., Tomita, T., Nakaya, K., Lee, VMY., Trojanowski, JQ., Iwatsubo, T. (1998). Aggregation of alpha-synuclein in Lewy bodies of sporadic Parkinson’s disease and dementia with Lewy bodies. American Journal of Pathology 152, 879-884.
Barnham, KJ., Masters, CL., Bush, AL. (2004). Neurodegenerative diseases and oxidative stress. Nature Reviews Drug Discovery 3, 205-214.
Bartels, T., Ahlstrom, L., Leftin, A., Kamp, F., Haass, C., Brown, M., and Beyer, K. (2010). The N-Terminus of the Intrinsically Disordered Protein α-Synuclein Triggers Membrane Binding and Helix Folding. Biophysical Journal 99, 2116-2124.
Braak, H., Del Tredici, K., Rub, U., de Vos, RAI., Steur, ENHJ., Braak, E. (2003). Staging of brain pathology related to sporadic Parkinson’s disease. Neurobiology of Aging 24, 197-211.
Braak, H., Ghebremedhin, E., Rub. U., Bratzke, H., Del Tredici, K. (2004). Stages in the development of Parkinson’s disease-related pathology. Cell and Tissue Research 318, 121-134.
Brandis, KA., Holmes, IF., England, SJ., Sharma, N., Kukreia, L., DebBurman, SK. (2006). Alpha-synuclein fission yeast model- Concentration-dependent aggregation without plasma membrane localization or toxicity. Journal of Molecular Neuroscience 28, 179-191.
Breydo, L., Wu, J., and Uversky, V. (2012). α-Synuclein misfolding and Parkinson’s disease. Biochimica Et Biophysica Acta (BBA) - Molecular Basis Of Disease 1822, 261-285.
Bungeroth, M., Appenzeller, S., Regulin, A., Völker, W., Lorenzen, I., Grötzinger, J., Pendziwiat, M., and Kuhlenbäumer, G. (2014). Differential aggregation properties of alpha-synuclein isoforms. Neurobiology Of Aging 35, 1913-1919.
Chartier-Harlin, MC., et. al. (2004). Alpha-synuclein locus duplication as a cause of familial Parkinson’s disease. Lancet 364, 1167-1169.
Chau, K., Ching, H., Schapira, A., and Cooper, J. (2009). Relationship between alpha synuclein phosphorylation, proteasomal inhibition and cell death: relevance to Parkinson’s disease pathogenesis. Journal Of Neurochemistry 110, 1005-1013
Chiti, F., Dobson, CM. (2006). Protein misfolding, functional amyloid and human disease. Annual Review of Biochemistry 75, 333-366.
Cuervo, AM., Stefanis, L., Fredenburg, R., Lansbury, PT., Sulzer, D. (2004). Impaired degradation of mutant alpha-synuclein by chaperone-mediated autophagy. Science 305, 1292-1295.
DebBurman, S., Wilcox, A. (2016). Primer Design. BIO 221 Molecules,Genes and Cells Laboratory Manuel (22-29) Lake Forest,IL: Lake Forest College
DebBurman, S., Wilcox, A. (2016). Step I: ɑ-Synuclein Gene Fragement Amplification by PCR BIO 221 Molecules,Genes and Cells Laboratory Manuel (30-31) Lake Forest,IL: Lake Forest College
DebBurman, S., Wilcox, A. (2016). Step II: Gene Fragement Purification by Gel Electrophoresis.BIO 221 Molecules,Genes and Cells Laboratory Manuel (32-37) Lake Forest,IL: Lake Forest College
DebBurman, S., Wilcox, A. (2016). Step III: Subcloning into a TOPO Vector and Bacterial Transformation. BIO 221 Molecules,Genes and Cells Laboratory Manuel (38-41) Lake Forest,IL: Lake Forest College
DebBurman, S., Wilcox, A. (2016). Step IV/V: Confirm Gene Fragment Orientation in Vector in Bacteria. BIO 221 Molecules,Genes and Cells Laboratory Manuel (42-51) Lake Forest,IL: Lake Forest College
DebBurman, S., Wilcox, A. (2016). Step VI: Plasmid Vector Transformation into Yeast. BIO 221 Molecules,Genes and Cells Laboratory Manuel (52-54) Lake Forest,IL: Lake Forest College
Dauer, W., Przedborski, S. (2003). Parkinson’s disease: Mechanisms and models. Neuron 39, 889-909.
Dawson, TM., Dawson, VL. (2003). Molecular pathways of neurodegeneration in Parkinson’s disease. Science 302, 819-822.
de Lau, LML., Breteler, MMB. (2006). Epidemiology of Parkinson’s disease. Lancet Neruology 5, 525-535.
Desplats, P., et. al. (2009). Inclusion formation and neuronal cell death through neuron-to-neuron transmission of alpha-synuclein. Proceedings of the National Academy of Sciences of the United States of America 106, 13010-13015.
Eliezer, D. (2013). The Mysterious C-Terminal Tail of Alpha-Synuclein: Nanobody’s Guess. Journal Of Molecular Biology 425, 2393-2396.
Fiske, M., Valtierra, et. al. (2011). Contribution of alanine-76 and serine phosphorylation in alpha-synuclein membrane association and aggregation in yeasts. Parkinson’s Disease 2011.
Giasson, B., Murray, I., Trojanowski, J., and Lee, V. (2000). A Hydrophobic Stretch of 12 Amino Acid Residues in the Middle of -Synuclein Is Essential for Filament Assembly. Journal Of Biological Chemistry 276, 2380-2386.
Gitler, AD., et. al. (2008). The Parkinson’s disease protein alpha-synuclein disrupts cellular Rab homeostasis. Proceedings of the National Academy of Sciences of the United States of America 105, 145-150.
Gorbatyuk, O., Li, S., Sullivan, L., Chen, W., Kondrikova, G., Manfredsson, F., Mandel, R., and Muzyczka, N. (2008). The phosphorylation state of Ser-129 in human -synuclein determines neurodegeneration in a rat model of Parkinson disease. Proceedings Of The National Academy Of Sciences 105, 763-768.
Haass, C., Selkoe, DJ. (2007). Soluble protein oligomers in neurodegeneration: lessons from the Alzheimer’s amyloid beta-peptide. Nature Reviews Molecular Cell Biology 8, 101-112.
Hejjaoui, M., et. al. (2012). Elucidating the role of c-terminal post-translational modifications using protein semisynthesis strategies: Alpha-synuclein phosphorylation at tyrosine 125. Journal of the American Chemical Society 134, 5196-5210.
Hendriks, I. and Vertegaal, A. (2016). A high-yield double-purification proteomics strategy for the identification of SUMO sites. Nature Protocols 11, 1630-1649.
Huh, WK., Falvo, JV., Gerke, LC., Carroll, AS., Howson, RW., Weissman, JS., O’Shea, EK. (2003). Global analysis of proteins localization in budding yeast. Nature 425, 686-691.
Kleinknecht, A., Popova, B., Lazaro, DF., Pinho, R., Valerius, O., Outeiro, TF., Braus, GH. (2016). C-terminal tyrosine residue modifications modulate the protective phosphorylation of serine 129 of alpha-synuclein in a yeast model of Parkinson’s disease. PLOS Genetics 12.
Kosten, J., et. al. (2014). Efficient modification of alpha-synuclein serine 129 by protein kinase CK1 requires phosphorylation of tyrosine 125 as a priming event. ACS Chemical Neuroscience 5, 1203-1208.
Krumova, P., et. al. (2011). Sumoylation inhibits alpha-synuclein aggregation and toxicity. Journal of Cell Biology 194, 49-60.
Lu, Y., Prudent, M., Fauvet, B., Lashuel, HA., Girault, HH. (2011). Phosphorylation of alpha-synuclein at Y125 and S129 alters its metal binding properties: Implications for understanding the role of alpha-synuclein in the pathogenesis of Parkinson’s disease and related disorders. ACS Chemical Neuroscience 2, 667-675.
McNaught, KS., Belizaire, R., Isacson, O., Jenner, P., Olanow,
CW. (2003). Altered proteasomal function in sporadic Parkinson’s disease. Experimental Neurology 179, 38-46.
Murray, I., et. al. (2003). Role of α-Synuclein Carboxy-Terminus on Fibril Formation in Vitro †. Biochemistry 42, 8530-8540
Nass, Richard.Przedborski, Serge. (Eds.) (2008) Parkinson’s disease :molecular and therapeutic insights from model systems Amsterdam; Elsevier/Academic Press, 3-48, 505-536.
Olanow, C. and Brundin, P. (2013). Parkinson’s Disease and Alpha Synuclein: Is Parkinson’s Disease a Prion-Like Disorder?. Movement Disorders 28, 31-40.
Oueslati, A. (2016). Implications of alpha-synuclein phosphorylation at S129 in synucleinopathies: What have we learned in the last decade?. Journal of Parkinson’s Disease 6, 39-51.
Ouslati, A., Paleologou, KE., Schneider, BL., Aebischer, P., Lashuel, HA. (2012). Mimicking phosphorylation at serine 87 inhibits the aggregation of human alpha-synuclein and protects against its toxicity in a rat model of Parkinson’s disease. Journal of Neuroscience 32, 1536-1544.
Outeiro, T. (2003). Yeast Cells Provide Insight into Alpha-Synuclein Biology and Pathobiology. Science 302, 1772-1775.
Ozansoy, M. and Başak, A. (2012). The Central Theme of Parkinson’s Disease: α-Synuclein. Molecular Neurobiology 47, 460-465.
Paleologou, KE., et. al. (2010). Phosphorylation at S87 is enhanced in synucleinopathies inhibits alpha-synuclein oligomerization, and influences synuclein-membrane interactions. Journal of Neuroscience 30, 3184-3198.
Park, S. (2002). Distinct Roles of the N-terminal-binding Domain and the C-terminal-solubilizing Domain of alpha -Synuclein, a Molecular Chaperone. Journal Of Biological Chemistry 277, 28512-28520.
Ristic, M., Brockly, F., Piechaczyk, M., and Bossis, G. (2016). Detection of Protein–Protein Interactions and Posttranslational Modifications Using the Proximity Ligation Assay: Application to the Study of the SUMO Pathway. Methods In Molecular Biology 279-290.
Rizzuto, R., Brini, M., Pizzo, P., Murgia, M., and Pozzan, T. (1995). Chimeric green fluorescent protein as a tool for visualizing subcellular organelles in living cells. Current Biology 5, 635-642.
Samuel, F., et. al. (2015). Effects of Serine 129 Phosphorylation on α-Synuclein Aggregation, Membrane Association, and Internalization. Journal Of Biological Chemistry 291, 4374-4385.
Sevcsik, E., Trexler, AJ., Dunn, JM., Rhoades, E. (2011). Allostery in a disordered protein: Oxidative modifications to alpha-synuclein act distally to regulate membrane binding. Journal of the American Chemical Society 133, 7152-7158.
Sharma, N., Brandis, KA., Herrera, SK., Johnson, BE., Vaidya, T., Shrestha, R., DebBurman, SK. (2006). Alpha-synuclein budding yeast model- Toxicity enhanced by impaired proteasome and oxidative stress. Journal of Molecular Neuroscience 28, 161-178.
Singleton, AB., et. al. (2003). Alpha-synuclein locus triplication causes Parkinson’s disease. Science 302, 841-841.
Sulzer, D., et. al. (2000). Neuromelanin biosynthesis is driven by excess cytosolic catecholamines not accumulated by synaptic vesicles. Proceedings of the National Academy of Sciences of the United States of America 97, 11869-11874.
Tenreiro, S., Hill, TF. (2010). Simple is good: Yeast models of neurodegeneration. FEMS Yeast Research 10, 970-979.
Tenreiro, S., et. al. (2014). Phosphorylation modulates clearance of alpha-synuclein inclusions in a yeast model of Parkinson’s disease. PLOS Genetics 10.
Thannickal, TC., Lai, YY., Siegel, JM. (2007). Hypocretin (orexin) cell loss in Parkinson’s disease. Brain 130, 1586-1595.
Uversky, V. and Lyubchenko, Y. (2013). Bio-Nanoimaging (Academic Press).
Valente, EM. et. al. (2004). Hereditary early-onset Parkinson’s disease caused by mutations in PINK1. Science 304, 1158-1160.
Vamvaca, K., Volles, M., and Lansbury, P. (2009). The First N-terminal Amino Acids of α-Synuclein Are Essential for α-Helical Structure Formation In Vitro and Membrane Binding in Yeast. Journal Of Molecular Biology 389, 413-424.