Creating a plasmid for the study of A30P and A53E mutations and the effects of decreased sumoylation in alpha-Synuclein in Parkinson’s
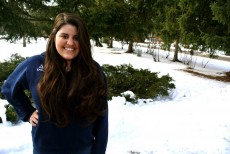
Sierra Smith & Lily Veldran
Department of Biology
Lake Forest College
Lake Forest, Illinois 60045
Download PDF
*This author wrote the paper as a part of BIOL221: Molecules, Genes, and Cell under the direction of Dr. DebBurman.
Abstract
Parkinson’s disease (PD) is a neurodegenerative disease that can be characterized by its negative affect on motor function. In PD there is aggregation of α-synuclein caused by either familial or sporadic mutations of the gene in the dopaminergic neurons of the substantia nigra. There are several familial mutations that are known to cause this aggregation in the cells, for example A30P, and several mutations that are newly discovered, such as A53E. One other factor thought to affect the solubility of α-synuclein is sumoylation. While these familial mutations and sumolyation have been studied separately, it is not known how they interact on the same gene. We hypothesize that when sumo is blocked through a mutation, K96R and K102R, α-Synuclein will increase aggregation. This study will be focused on transforming a plasmid into yeast that will be able test both the familial mutations A30P and A53E coupled with a mutation that blocks sumolyation. Two gene fragments containing these mutations were successfully subcloned into the pYES2.1 TOPO E.coli vector and checked for correct orientation. Orientation was confirmed through whole cell and plasmid based PCR and a gene sequencing analysis. The plasmid vector for the A30P mutation was not successfully transformed into yeast but A53E plasmid vector was successfully transformed. These mutations, now expressed in yeast, will allow for future studies to be completed that may increase knowledge of Parkinson’s disease as a whole and how SUMO mutations in these two familial α-synuclein mutations affect aggregation and disease pathology.
Introduction
Parkinson’s disease (PD) is a neurodegenerative disease that affects motor function. More common in older adults, around the age of 60, PD usually presents itself first in the form of tremors on one side of the body. These tremors gradually worsen into other symptoms that spread to both the right and left side of the body. Other common symptoms include a combination of rigidity, bradykinesia, and loss of postural reflexes (Fahn, 2011). PD comes in two varieties, sporadic and familial. Sporadic is more common and characterized by general occurrences in the population. Familial is less common and is inherited from a family member.
In the brain, PD affects the substantia nigra in which there is a striatal dopamine deficiency due to loss of dopaminergic neurons in the substantia nigra. In the early stages of the disease, the medulla oblongata is affected first then the basal ganglia. A post-mortem examination of a patient’s brain shows that there is the presence of Lewy bodies, or cytoplasmic inclusions, consisting of a protein called α-Synuclein in the damaged cells.
α-Synuclein is a 140 amino acid soluble protein. It has 3 distinct regions, N, M, and C. The N-terminal region has repeating sequences that relate to a lipid-binding motif, which helps protein binding to phospholipid vesicles. The central region, the M region, is extremely hydrophobic, and has been implicated in forming amyloid fibrils. The C-terminal region is hydrophilic and rich in proline, glutamate, and aspartate. It helps with chaperone activity to α-Synuclein. α-Synuclein does not have a secondary or tertiary structure; it is an unfolded protein because it mainly acts as a chaperone. (Irvine, El-Agnaf, Shankar, & Walsh, 2008). In PD mutant α-Synuclein forms aggregates and increases PD pathology. However, mutations in α-Synuclein only account for a small percentage of PD cases.
There are several known α-Synuclein mutations that cause Familial PD such as A30P (Kruger, et al. 1998), E46K (Zarranz, et al. 2003), H50Q (Ghosh, 2013), G51D (Lesage, 2013), A53E (Pasanen, 2014), and A53T (Polymeropoulos, et al. 1997). The familial mutants focused on in this study are A30P and A53E. A30P is a better-known mutation, in which the Arginine at the thirtieth amino acid is mutated to Praline. A53E is more newly discovered and has not been studied in depth; the Arginine at the fifty-third amino acid is mutated to Glutamic Acid (Ghost, 2014).
SUMO is a protein that binds to α-Synuclein to aid in solubility and prevent aggregation. Sumoylation, the process that is initiated by the binding of SUMO to a protein, helps to regulate aggregation in a cell by increasing protein solubility. SUMO proteins are a family of small proteins that are covalently attached to and detached from other proteins in cells to modify their function (Hay, 2005). SUMO proteins are similar to ubiquitin, and ate directed by an enzymatic cascade analogous to that involved in ubiquitination. However, instead of tagging a protein, SUMO matures, then cleaves its last four amino acids to bind with a lysine (Dorval & Fraser, 2006).
In this study, there is a mutation in the C terminus of both α-Synuclein familial mutations that affects SUMO binding and prevents sumoylation. The lysines at the 96th and 102nd amino acid, that is normally where SUMO binds, are replaced with arginines. This mutation does not allow sumoylation to occur, potentially causing aggregates of α-Synuclein to form. These aggregates are characteristic of Parkinson’s (Krumova, et al., 2011).
It is known that sumoylation improves solubility, and that the mutated form of the gene causes aggregation. It is not known how the sumo mutation and the familial mutation interact and affect Parkinson’s pathology. We hypothesize that when SUMO is blocked through the mutation, α-Synuclein will increase aggregation.
Yeast will be used to test this hypothesis. Yeast or Saccharomyces cerevisiae is a good model organism because it has a short generation time and can grow on a variety of media. This makes it readily available and can provide an invaluable starting point for later analysis in more complex organisms. This organism can be easily transformed with extra-chromosomal plasmids or by genomic insertion.
In the context of studying Parkinson’s disease, yeast can express ɑ-Synuclein and any mutants of α-Synuclein in the same way as humans do as they make and fold proteins in the same way. If a mutated plasmid vector is inserted into yeast and then expressed as a protein, the yeast can be studied for the affects of the mutant protein. In this study, the yeast with mutated forms of α -Synuclein and the sumoylation mutations will be looked at for any effects. These results are compared to yeast with the wild type form of α-Synuclein, which are normally functioning. From this experiment, we can see how yeast can control their cells when their cellular environment becomes toxic (Outeiro, & Lindquist, 2003). The aim of this study is to take two plasmids containing the mutated α-Synuclein gene fragments with SUMO mutations, and tagged with GFP, and to transform them into E.coli, purify and analyze them, and then finally transform them into yeast. We first will amplify the familiar mutants A53E and A30P α-Synuclein with the additional K96R and K102R SUMO mutations, which are also fused C-terminally with GFP and a V-5 His tag from a mammalian cell culture plasmid. The fragment will then be put into a plasmid vector and transformed into E. coli. The subcloned vector will then be checked for correct orientation using whole cell PCR and plasmid prep PCR. The plasmid will then put into an expression vector and amplified in bacteria. After selecting the correct orientation of DNA in the vectors and purifying them from bacteria. These vectors will then be transformed into yeast and grown on plates to be used in later experiments.
Results
Gene Fragment Amplification by PCR
The first step in the procedure for developing a yeast model (Figure 1C) with A30P and A53E mutations was to amplify the genes from a plasmid created earlier. In the plasmid the mutated gene was linked with GFP and a V5 a His tag to aid in the later study of Parkinson’s (Figure 1A). We used PCR (polymerase chain reaction) and the appropriate forward and reverse primers (Figure 1B) in order to amplify the gene. To ensure that some product was successfully amplified different amounts of plasmid were used for a total of 7 PCR reactions including positive and negative controls (Table 1). See methods section for complete details.
Gene Fragment Purification by Gel Electrophoresis
In order to tell if there was PCR product, gel electrophoresis was run with each of the 7 PCR reactions. Two gels were run, one specifically checking for purification of the two specific gene fragments, Gel 1, and one checking for PCR product against the positive and negative controls, Gel 2. We predicted the bands containing the fragments (Figure 2A) to be near 1185 base pairs because of calculations made by looking at the length of the α-synuclein gene plus the length of GFP attached. The actual gel showed that the bands were in the predicted positions (lanes 3-6, Gel 1) and that the controls (lanes 4, 5, & 8, Gel 2) worked (Figure 2B). In Gel 1 the bands were extremely faint underneath UV light and it was unclear whether there was PCR product of A30P and A53E.
To purify the DNA from the gels, the bands were cut out. However, because the bands were faint, it was more difficult to separate the product from the gel and be sure that the bands were fully cut out. We imaged the gel after the bands were cut to compare it to the first gel picture (Figure 2C). Upon comparison of the two gel pictures (before and after DNA removal) it looked like the entirety of the bands were properly removed and all of the DNA produced from PCR was retrieved. The DNA was separated from the gel by addition of a salt solution and run on a gel to check that purified DNA was recovered (Figure 2D). See ‘PCR purification’ in the methods section for complete details. This step will allow us to identify if there was any PCR product.
Subcloning into Plasmid Vector and Bacterial Transformation
The next step was to subclone the purified DNA fragments into a plasmid vector and transform that vector into bacteria. The purpose of transforming the plasmid into bacteria before yeast is to test if the DNA fragments were cloned into the plasmid vector in the correct orientation before they are inserted into the yeast model. In this step E.coli also multiply the plasmid for isolation to put into yeast.
The two α-synuclein fragments were sub cloned into the TOPO-pYES2 vector and then added to TOP10 One Shot® Chemically Competent E. coli. The E. coli were spread onto LB+AMP plates, grown overnight, and evaluated for growth the next day. The control plates grew as expected, no growth on negative controls and growth on the positive control. There was very minimal growth on the plates with A30P and A53E fragments (Figure 3E). From the few individual colonies, four were chosen from each plate and transferred into LB+AMP liquid culture tubes. These tubes were then grown overnight to be used later for Plasmid-based PCR. See ‘Vector Subcloning and Bacterial Transformation’ in the methods section for complete details.
Orientation check I: Whole Cell PCR and Plasmid Prep + PCR
Next, we actually test the orientation of the gene fragments in the vector by PCR analysis. The vector could have inserted in two ways, with only one being aligned correctly for expression. To identify which colonies marked previously might have the correct orientation, we used the successful transformation reaction from the previous step and performed whole cell and plasmid based PCR. The PCR reaction will also amplify the gene region of interest. In both PCR reactions the Gal Forward primer was used because it binds to the gene promoter along with a reverse primer that binds to the 3’-end of the gene fragments. There will be no PCR product if the gene is in the incorrect orientation, and product if the orientation is correct.
For plasmid based PCR, the plasmid first needs to be extracted from the E. coli in order to be inserted into other organisms. We used an alkaline treatment to gently lyse the E. coli cells that had grown in the liquid LB+AMP liquid overnight and extract the plasmid. The E. coli grown on plates was used for whole cell PCR. 17 PCR tubes were created and run, 8 with the fragment A30P, 8 with the fragment A53E, and one control plasmid. To show that the plasmid preparation was correct a gel was run using each sample of plasmid prepared for both fragments (Figure 4C). See ‘Bacterial Whole Cell PCR’ and ‘Plasmid Purification’ in the methods section for complete details.
Orientation check II: Whole Cell and Plasmid PCR Gel Electrophoresis
In this step, the PCR product from the previous whole cell and plasmid PCR will be analyzed by gel electrophoresis. Two gels were run, one looking at fragments A30P and the other looking at fragment A53E. If the orientation is correct there will be a band at around 1100 bp (Figure 4A & Figure 5A). In the gel run with fragment A30P there were two dark bands from the plasmid based PCR product of colonies 2 and 4 (lanes 4 and 6). The whole cell PCR didn’t produce any bands (lanes 7-10) (Figure 4B). We chose these plasmids, from colonies 2 and 4, to send to the University of Chicago for Sequencing. In the gel run with fragment A53E there was one dark band and a very faint one from the plasmid based PCR product of colonies 1 and 4 (lanes 3 and 6). Whole cell PCR, again, didn’t produce any bands (lanes 7-10) (Figure 5B). We chose the plasmid from colonies 1 and 4 to send for sequencing. See ‘DNA Sequencing’ in the methods section for complete details.
Plasmid Vector Transformation into Yeast
In the last step we will introduce the correctly oriented plasmids into BY4741 yeast. Because yeast are fungi and have a cell wall, we used lithium acetate (LiAc) to permeate the cell wall and allow for the uptake of the two plasmid vectors. The samples were plated on SC-URA glucose, using either 30 μL or 200 μL samples. The plates were then placed into a 30°C incubator and grown for five days. Several YPD plates were used as a control (Figure 7). The plates of yeast that transformed fragment A53E had growth with both 30 μL and 200 μL samples. The plates with yeast transformed with fragment A30P had no growth. The positive and negative controls were successful with growth on the positive and no growth on the negative (Figure 7).
Discussion
The purpose of this study was to look at two α-synuclein fragments each with a familial Parkinson’s mutation, A30P and A53E, and two SUMO mutations, K96R and K102R, and transform them into a yeast vector. A53E transformed successfully and A30P did not transform successfully.
We successfully purified and extracted the A30P and A53E gene fragments from the original plasmid through PCR amplification and gel electrophoresis purification. The purification was seen to be successful because the purified bands appeared at the expected length of about 1185 base pairs. While at the correct location, the bands were extremely faint and extra care had to be taken to remove all of the DNA. The bands might have been faint because the PCR reaction was not as effective as it could have been due to an error in the reaction mixture or a defective ‘ingredient’. Once the gene fragments were extracted, the gel was reimaged and showed that the DNA was no longer in the gel meaning all of it was successfully extracted. A gel run with the products extracted also showed that DNA was successfully extracted, as all of the bands were present at about 1185 base pairs.
The next step was subcloning the purified fragment into a pYES2.1 TOPO vector and transforming it into E. coli. We expected that a successful transformation would result in E. coli growth on LB+ampicillin plates because there is an ampicillin resistant gene in the TOPO vector. The E. coli without successful transformation will not survive on LB plates with ampicillin present because they did not receive the ampicillin resistant gene from the vector.
The 30 μL transformation was unsuccessful for both A30P and A53E because no bacteria grew. This could be because of the faint bands from the previous purification and extraction step or simply because a lower number of E. coli were spread on the plate with only a 30 μL sample leaving a smaller chance that there were some that had successfully transformed. However, on the 200 μL plates, there was individual colony growth for both the A30P and A53E fragments. This indicates that there was successful insertion of the purified DNA fragments into the TOPO vector and successful transformation of that vector into E. coli.
To check if the A30P and A53E had been entered into the TOPO vector in the correct orientation for expression, four colonies were selected from each successful fragment transformation. In running a gel of whole cell and plasmid based PCR using E.coli from each of the chosen colonies, we expect to see a band at around 1185 base pairs as that is the length of the fragments. If there is no band at this length then the DNA fragment was inserted in the incorrect orientation. The forward primers used were designed to bind to the gene promoter and the reverse primer used binds to the 3’-end of the gene fragments. There will be no PCR product if the gene is in the incorrect orientation, and product if the orientation is correct because the primers will only be able to bind to a fragment with correct orientation.
Plasmid based PCR of fragment A30P showed two bands at the correct length, meaning that both of those colonies had transformed a vector in which the DNA fragment had been inserted in the correct orientation. Whole cell based PCR of fragment A30P was either unsuccessful or there were no colonies with correctly oriented fragments, there are no bands of any length in the gel. Because there are no bands at all in the gel for whole cell based PCR, it is likely that the PCR reaction was unsuccessful, this could be because of the small amount of cells being used or a mistake in creating the reaction mixture.
Plasmid based PCR of fragment A53E showed two bands at the correct length, meaning that they have transformed a vector in which the fragment is in the correct orientation. Again, whole cell based PCR of A53E was unsuccessful, showing no bands of any length in the gel. This suggests that it is more likely that there was a problem in the preparation of the whole cell PCR reactions because both fragments did not have any bands through whole cell PCR. The purified plasmid was checked and confirmed as is shown in a gel where all of the colonies chosen for plasmid have bands in the correct places at around 1100 base pairs.
To confirm the positive results of the plasmid based PCR, we sent out the correctly oriented products from each fragment for sequencing. When compared to the known sequence of our mutated α-synuclein fragments, their orientation was confirmed.
The final step was to isolate the correctly oriented plasmids from E.coli and insert them into yeast. The plasmids used were previously purified before being run through plasmid based PCR to check for orientation. The PYES2.1 TOPO vector contains a healthy copy of the URA3 gene, meaning that yeast cells that successfully transform the TOPO vector will be able to survive on media not supplemented with uracil. On SC-URA plates, plates deficient in uracil, we expect to see growth of only yeast that have successfully transformed the plasmid vector.
Yeast transformed with the plasmid vector containing A53E was successful. There was growth on all plates, whether plated with 30 μL or 200 μL samples. The growth shows that the yeast are able to survive without uracil and therefor have the URA3 gene provided by the vector with the A53E fragment inserted into it.
Yeast transformed with plasmid vector containing A30P was not successful. There was no growth on any of the plates, whether plated with 30 μL or 200 μL samples. The positive and negative controls were successful showing that the yeast cell line is not the problem. On YPD plates, on which the URA3 gene is not required for yeast survival, yeast grew in all conditions showing that the BY4741 yeast used were growing correctly. A potential issue could have been a mistake in the transformation procedure that prevented the cell walls in the yeast from being broken down. If the cell walls were not perforated correctly, the plasmid vector would not be able to transform into the yeast simply because it could not get into the cells.
Some future studies that can be done with these mutated gene fragments in yeast include seeing how α-synuclein targets the plasma membrane, and induces toxicity in yeast. This can be done using fluorescent microscopy to see where the genes are expressed in the cell using GFP, or other fluorescent staining (Dixon, et al. 2005). Another experiment could be depleting Phosphatidylethanolamine in yeast to see how homeostasis of α-synuclein is affected. By staining for specific parts of a cell, you can merge images to see cell growth under stress and see if there are any significant findings from theses merged images (Wang, et al. 2014). A third study that can be done is seeing how α-synuclein can be modulated. This can be done by a not so common technique of creating different gradients for the cells to react to. This technique can show changes in toxicity levels of α-synuclein in different environments (Fernandes, et al. 2014). A final experiment that can be used with α-synuclein fragments in yeast models can be seeing if α-synuclein can bind to lipid droplets. The composition of the lipid droplets to see if the fragment binds can be done using mass spectroscopy (Wang. S, et al. 2013).
In conclusion, the transformation of the A53E plasmid into yeast was successful and the transformation of the A30P plasmid was not. These mutations, now expressed in yeast, will allow for studies to be completed that may increase knowledge of Parkinson’s disease as a whole and how a block of sumoylation in these two familial α-synuclein mutations affect aggregation and disease pathology.
Materials and Methods
Primer design and synthesis:
The PCR primers designed were each 30 nucleotides long. To design the forward primer we looked at the 5’ end of the α-synuclein coding sequence, the primer is the first 30 nucleotides of the coding strand. To design the reverse primer we looked at the 3’ end of the GFP tag added on to the end of the α-synuclein coding sequence, and counted 30 nucleotides in the 5’ to 3’ direction of the template strand.
(DebBurman, 2013, 25-26)
Template DNA, sub cloning plasmids, and bacterial and yeast cell used:
The control plasmid used was GFP-A30P (1-110). α-synuclein has been truncated at the C-terminus and is only 110 base pairs long. This α-synuclein also has the A30P mutation, and it has GFP tagged at the N-terminus, instead of the C-terminus. The subcloning plasmid used was pYES2.1/V5- His-TOPO. This vector has ampicillin resistance and a healthy copy URA3 gene (DebBurman, 2013, 27). The bacterial cells used were TOP10 One Shot ® Chemically Competent E. coli (DebBurman, 2013, 39). The yeast cells used were s. cerevisiae BY4741. (DebBurman, 2013, 53)
Plasmid-based PCR
9 Plasmid based PCR reactions were performed. 4 from fragment A30P, one reaction from each colony selected, and 4 from fragment A53E. A positive control was made using the original plasmid.
Primer stock of 33X:
-25 μL of Master Mix (MgCl2, Buffer, Taq DNA Polymerase, dNTP)
-21 μL of Sterile RNase-free H2O.
-1.5 μL of Gal Forward Primer
-1.5 μL of GFP Reverse Primer-1 μL of plasmid (purified beforehand, see plasmid purification method)
Run the PCR machine, [95°C for 30 seconds, 55°C for 30 sec, 72°C for 30 sec] x 29. 72°C for 30 minutes and then store the PCR reaction tubes at 4°C. (DebBurman, 2013, 49)
PCR purification
To separate and purify amplified gene fragments, gel electrophoresis was run with each PCR product. The appropriate bands were manually cut out and purified through the use of GENECLEAN Turbo Kit. Gels were imaged before and after DNA extraction and the weight of centrifuge tube recorded before extraction. The gels were placed on a UV box to show fluorescent PCR bands. DNA fragments were taken out with a clean scalpel, cut directly above and below the desired band. Gel slices placed in centrifuge tube and weighed again to find the weight of the gel. 100 μL of turbo salt solution was added per .1 g of gel slice and incubated at 55°C for 10 minutes or until gel has dissolved. Centrifuge at 13,000x for 30 seconds and discard the liquid in the catch tube. Add 500 μL of turbo wash/ethanol solution and centrifuge for 4 minutes. Add 30 μL of the turbo elution solution and incubate for 5 minutes at room temperature. Centrifuge at 13,000x for one minute. (DebBurman, 2013, 34-36; QIAGEN QIAquick Gel Extraction Kit, 23)
Vector Subcloning and Bacterial Transformation
To subclone the purified plasmid into a vector several reaction tubes were created, 1 using A30P plasmid and 1 using A53E plasmid. In each tube there was 4 μL of purified PCR product, 1 μL salt solution, and 1 μL of TOPO vector. The subcloning vector used was pYES2.1/V5-His-TOPO. This vector has ampicillin resistance and a healthy copy URA3 gene. (DebBurman, 2013, 27; pYES2.1 TOPO® TA Expression Kit). Incubate for five minutes at room temperature. Using 1 μL of water instead of the TOPO vector created a negative control.
To transform the newly subcloned vectors into s. cerevisiae PY4741, 2 μL of each TOPO® Cloning reaction was added to two different vials of TOP10 One Shot® Chemically Competent E. coli and mixed. This mixture is incubated on ice for 5 minutes and then heat shocked at 42°C for 30 seconds. 250 μL of SOC medium was added to the mixture, cap and shake tube for 1 hour horizontally. Create 8 plates of LB+AMP medium and plate 200 μL and 30 μL of each fragment, along with the positive transformation control, negative transformation control and the cloning control. The growth of the bacterial colonies was checked 24 hours later and 4 colonies from each fragment were marked; a sample of each of the chosen colonies was transferred to liquid LB+AMP medium. (DebBurman, 2013, 39)
Bacterial Whole Cell PCR
A total of 8 PCR reactions were created using whole cell PCR, 4 from the selected colonies of E. coli with the vector containing A30P, and 4 from the selected colonies of E. coli with the vector containing A53E. The same positive control was used as in plasmid based PCR.
Primer stock of 33X:
-25 μL of Master Mix (MgCl2, Buffer, Taq DNA Polymerase, dNTP)
-21 μL of Sterile RNase-free H2O.
-1.5 μL of Gal Forward Primer
-1.5 μL of GFP Reverse Primer
-1 μL of plasmid (taken directly from plates by lightly touching the selected colony with a toothpick and introducing the sample to the solution)
Run the PCR machine, [95°C for 30 seconds, 55°C for 30 sec, 72°C for 30 sec] x 29. 72°C for 30 minutes and then store the PCR reaction tubes at 4°C. (DebBurman, 2013, 42)
Plasmid Purification
To prepare for plasmid based PCR, we used protocol designed to isolate bacterial plasmid using the QIAGEN QIAprep® Miniprep Kit (QIAGEN QUIAprep®, 22). E. coli bacteria grown over night in LB+AMP liquid media over night was centrifuged to pellet the cells. 250 μL of Buffer P1 was added and the pellet suspended, 250 μL of Buffer P2 was added and mixed into the solution. 350 μL of Buffer N3 as added and the solution mixed until no longer blue in color. Centrifuge for 10 minutes and transfer the supernatant into a QIAprep column. Wash using Buffer PB and elute by using Buffer EB. (DebBurman, 2013, 48)
DNA sequencing
The gene fragments were sent to the University of Chicago DNA Sequencing & Genotype Facility. The method used by this lab is the chain termination method (DebBurman, 2013, 50).
Yeast transformation
The yeast being used is s. cerevisiae BY4741 because they have disrupted function of the URA 3 gene. Cells were centrifuged at 14,000xg for 30 seconds and the latent LiAc was removed. Add 200 μL of 100 mM LiAc and vortex to resuspend the cells. Separate out solution into 40 μL samples in microcentrifuge tubes. Centrifuge cells again and remove LiAc with a pipette. Add transformation mix to each of the tubes. Transformation mix:
-240 μL of PEG (50% w/v)
-36 μL of 1.0 M LiAc
-25 μL of single-stranded carried DNA (2.0 mg/mL)
-46 μL of H2O
-5 μL of plasmid DNA
Vortex each tube until the pellet is completely mixed. Incubate for 30 minutes at 30°C. Heat shock for 25 minutes in 42°C water bath. Centrifuge at 6,000x for 30 seconds and remove the transformation mix. Add 1 mL of water to each tube and mix carefully. 10 sample were plated on SC-URA media, two 200 μL and two 30 μL of each fragment plus the positive and negative controls. 6 YPD plates were plated with each concentration of each fragment and both controls. (DebBurman, 2013, 53-55)
Acknowledgements
Special thanks to the Lake Forest College Department of Biology, Dr. DebBurman, Dr. Wilcox, Alex Roman and Khadijah Hamid for providing valuable resources in the writing of this paper.
References
Bishop, N.A., Lu, Tao., and Yankner, B.A. (2010). Neural mechanisms of ageing and cognitive decline. Nature. 464, 529-535
Bourdenx, M., Bezard, E., and Dehay, B. (2014). Lysosomes and -synuclein form a dangerous duet leading to neuronal cell death. Front Neuroanat. 8, 83.
Breydo, L., Wu, J.W., and Uversky, V.N. (2012).α-Synuclein misfolding and parkinson’s disease. Biochim Biophys Acta. 1822, 261- 285.
Cherry, J.M., Hong, E.L., Amundsen, C., Balakrishnan, R., Binkley, G., Chan, E.T., Christie, K.R., Costanzo, M.C., and Wong, E.D. (2012). Saccharomyces genome database: the genomics resource of budding yeast. Nucleic Acids Res. D700-5, 1-6.
Cuervo, A.M., Stefanis L., Fredenburg, R., Lansbury, P.T., and Sulzer, D. (2004). Impaired degradation of mutant α-synuclein by chaperone-mediated autophagy. Science. 305, 1292-5.
DebBurman, S. (2013). Primer Design. In S. DebBurman (Ed.), BIO221 molecules, genes, & cells laboratory manual (pp. 25-26). Lake Forest, IL: Lake Forest College.
DebBurman, S. (2013). Gene Fragment Purification by Gel Electrophoresis. In S. DebBurman (Ed.), BIO221 molecules, genes, & cells laboratory manual (pp. 31-36). Lake Forest, IL: Lake Forest College.
DebBurman, S. (2013). Subcloning into Plasmid Vector and Bacterial Transformation. In S. DebBurman (Ed.), BIO221 molecules, genes, & cells laboratory manual (pp. 37-40). Lake Forest, IL: Lake Forest College.
DebBurman, S. (2013). Confirmation Gene Fragment Orientation
in Vector in Bacteria. In S. DebBurman (Ed.), BIO221 molecules, genes, & cells laboratory manual (pp. 41-46). Lake Forest, IL: Lake Forest College.
DebBurman, S. (2013). Plasmid Vector Purification and Sequencing. In S. DebBurman (Ed.), BIO221 molecules, genes, & cells laboratory manual (pp. 47-51). Lake Forest, IL: Lake Forest College.
DebBurman, S. (2013). Plasmid Vector Transformation into Yeast. In S. DebBurman (Ed.), BIO221 molecules, genes, & cells laboratory manual (pp. 53-55). Lake Forest, IL: Lake Forest College.
Dixon, C., Mathias, N., Zweig, R.M., Davis, D., and Gross, D. (2005). α-Synuclein targets the plasma membrane via the secretory pathway and induces toxicity in yeast. Genetics. 170. 47-59.
Dorval, V., Fraser, P.E. (2006) Small ubiquitin-like modifier (SUMO) modification of natively unfolded proteins tau and α-synuclein. J Biol Chem. 281, 9919-9924.
Fernandes, J.T., Tenreiro, S., Gameiro, A., Outeiro, T.F., and Conde, J.P. (2014). Modulation of alpha-synuclein toxicity in yeast using a novel microfluidic-based gradient generator. Lab Chip. 14, 3949-57.
Ghosh, D., Mondal, M., Mohite, G.M., Singh, P.K., Ranjan, P., Ghosh, S., Jha, N.N., Kumar, A., and Maji, S.K. (2013). The Parkinson’s disease-associated H50Q mutation accelerates α-Synuclein aggregation in vitro. Biochemistry. 52, 6925-7.
Ghosh, D., Sahay, S., Ranjan, P., Salot, S., Mohite, G.M., Singh, P.K., Dwivedi, S., Carvalho, E., Banerjee, R., Kumar, A., and Maji, S.K. (2014). The newly discovered parkinson’s disease associated finnish mutation (A53E) attenuates α-synuclein aggregation and membrane binding. (2014). Biochemistry. 53, 6419-21.
Hay, R.T. (2005). SUMO: a history of modification. Mol. Cell. 18, 1–12.
Kruger, R., Kuhn, W., Muller, T., Woitalla D., Graber, M., Kosel, S., Przuntek, H., Epplen, J., Schols, L. & Riess, O. (1998). AlaSoPro mutation in the gene encoding α-synuvlein in Parkinson’s disease. Nat Genet. 18, 106-108.
Krumova, P., Meulmeester, E., Garrido, M., Tirard, M., Hsiao, H., et. al. (2011). Sumoylation inhibits α-synuclein aggregation and toxicity. J Cell Biol. 194, 49-60.
Lesage, S., Anheim, M., Letournel, F., Bousset, L., Honoré, A., Rozas, N., Pieri, L., Madiona, K., Dürr, A., Melki, R., Verny, C., and Brice, A. (2013) G51D α-synuclein mutation causes a novel parkinsonian-pyramidal syndrome. Ann Neurol. 73, 459-71.
Marxreiter, F., Nuber, S., Kandasamy, M., Klucken, J., Aigner,
R., Burgmayer, R., Couillard-despres, S., Riess, O., Jurgen, W. & Winner, B. (2009). Changes in adult olfactory bulub neurogenesis in mice expressin the A30P mutant form of alpha-synuclein. Eur J Neurosci. 29, 879-890.
Outeiro, T.F. and Lindquist, S. (2003). Yeast cells provide insight into alpha-synuclein biology and pathobiology. Science. 302, 1772-1775.
Pasanen, P., Myllykangas, L., Siitonen, M., Raunio, A., Kaakkola S., Lyytinen, J., Tienari, P.J., Pöyhönen, M., and Paetau A. (2014). A novel α-synuclein mutation A53E associated with atypical multiple system atrophy and Parkinson’s disease-type pathology. Neurobiol Aging. 35, 2180-5
Pimentel, C., Batista-Nascimento, L., Rodrigues-Pousada, C.,
Menezes, R.A. (2012). Oxidative stress in alzheimer’s
and Parkinson’s diseases: insights for the yeast saccharomyces cerevisiae. Oxid Med Cell Longev. 2012, 1-9.
Polymeropoulos, M.H., Lavedan, C., Leroy, E., Ide, S.E., et
al…., and Nussbaum, R.L., (1997). Mutation in the α-synuclein gene identified in families with parkinson’s disease.
276, 2045-2047.
pYES2.1 TOPO® TA Expression Kit, (2004) . Version J (pp. 1-3, 7-10, 18-19).
QIAGEN QIAprep® Miniprep Kit (250) [Cat. No. 27016] Page 22, QIAprep Miniprep Handbook: QIAprep Spin Miniprep Kit Protocol.
QIAGEN QIAprep® Miniprep Kit (250) [Cat. No. 28704] Page 23, QIAprep Miniprep Handbook: QIAprep Gel Extractoin Kit.
Sousa, V.L., Bellani, S., Giannandrea, M., Yousuf, M., Valtora, F., Meldolesi, J. & Chiergatti, E. (2009). α-synucein an
Its A30P Mutant affect actin cytoskeletal structure and dynamics. Mol Biol Cell., 20, 3725-3739.
Wang, S., Horn, P.J., Liou, L.C., Muggeridge, M.I., Zhang, Z., Chapman, K.D., and Witt, S.N. (2013). A peroxisome biogenesis deficiency prevents the binding of alpha- synuclein to lipid droplets in lipid-loaded yeast. Biochem Biophys Res Commun. 438, 452-456.
Wang, S., Zhang, S., Liou, L.C., Ren, Q., Zhang, Z., Caldwell, G.A., Caldwell, K.A., and Witt, S.N. (2014.
Phosphatidylethanolamine deficiency disrupts α-synuclein homeostasis in yeast and worm models of Parkinson disease. Proc Natl Acad Sci U S A., 111, E3976-85.
Zarranz, J.J., Alegre, J., Gomez-Esteban, J.C., Lezcano, E., et al…, and de Yebenes, J.G. (2004). The new mutation, E46K, of alpha-synuclein causes Parkinson and Lewy body dementia. Ann Neurol. 55, 164-173.
FIGURES:
Figure 1. Overall Project Design. (A) Alpha-synuclein cartoon Wild type vs. A30P fragment and A53E fragment being studied. (B) PCR primers (C) Overall schematic of experimental design. Step 1 completed by Alex Roman beforehand, step 2-7 to be done in this study.
Table 1. PCR primer design table. Each PCR tube was prepared with the specified amount of master mix, forward primer, reverse primer, plasmid, and water, then put through PCR. Reference this table when looking at Figure 2.
Figure 2. (A) Computerized ideal gel of gene fragment Purification. Gel 1 should have bands at abut 1100 bp for wells labeled Tube 2, 1, 5, &6. Gel 2 should have bands at 1100 for wells labeled tube 1, 2, 5, 6, & 7, and bands near the well for tubes 3 &4, the negative controls. (B) PCR gel photograph before removal of DNA. There were bands near 1100 bp as expected in Gel 1. Gel 2 did not run as expected, only tube 7 had the expected result. (C) PCR photograph after the removal of DNA. The bands previously at ~1100 bp were cut out successfully.
Figure 3. Picture of E. coli transformation plates. (A) E.coli, LB + AMP, 10/3/14, PYES2, A30P, 200 μL shows minimal growth. Selected colonies circled for future testing. (B) E.coli, LB + AMP, 10/3/14, PYES2, A53E, 200 μL shows minimal growth. Selected colonies circled for future testing. (C) E.coli, LB + AMP, 10/3/14, PYES2, A30P, 30 μL and E.coli, LB + AMP, 10/3/14, PYES2, A53W, 30 μL show no bacterial growth. (D) Positive transformation control shows growth. Negative transformation control shows no growth. Negative Cloning control shows no growth.
Figure 4. Orientation Check II: Fragment A30P. (A) Computerized ideal gel with in which all tested colonies (see fig 3A) have correct orientation and plasmid based and whole cell PCR are successful. (B) Actual gel photograph of the A30P fragment gel. Whole cell PCR was unsuccessful, there are no bands. Plasmid based PCR was successful and shows two colonies with the correct orientation (shown with red circles). These two colonies, 2 and 4, are selected for further study.
Figure 5. Orientation Check II: Fragment A53E. (A) Computerized ideal gel with in which all tested colonies (see fig 3B) have correct orientation and plasmid based and whole cell PCR are successful. (B) Actual gel photograph of the A53E fragment gel. Whole cell PCR was unsuccessful, there are no bands. Plasmid based PCR was successful and shows two colonies with the correct orientation (shown with red circles). These two colonies, 1 and 4, are selected for further study
Figure 6. Picture of Yeast Transformation Plates. (A) SC-URA plated with yeast transformed with fragment A53E. Growth on the plates indicates that the transformation was successful. (B) SC-URA plated with yeast transformed with fragment A30P. No growth on the plates indicates that the transformation was not successful, though the positive and negative control were successful. (C) YPD control plates show that the s. cerevisiae PY4741 yeast were growing efficiently.