Co-regulation of miRNA biogenesis and alternative pre-mRNA splicing of host gene
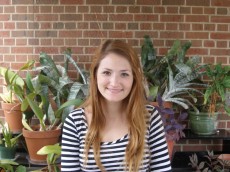
Ashley A. Reich
Department of Biology
Lake Forest College
Lake Forest, Illinois 60045
Download PDF
Abstract
This study focuses on two post-transcriptional gene expression regulatory mechanisms found ubiquitously in the body and widely expressed in neuronal tissue: host gene pre-messenger RNA (pre-mRNA) alternative splicing and microRNA (miRNA) biogenesis. Most of the past research has primarily looked at how miRNAs regulate gene expression at the pre-mRNA level, and little has been done on how these two processes coexist. We hypothesized that the two processes are co-regulated when the miRNA is located within the intron of a pre-mRNA, and that miRNA biogenesis impacts the inclusion of alternative exons in the final mRNA transcript. Our results revealed that manipulations to both processes had effects on the other process. We conclude that some level of co-regulation exists between these two processes, and that the Microprocessor may have some role in alternative splicing.
Introduction
Diversity of gene expression regulation
Each cell produces many different proteins in order to differentiate itself from other cells and to function properly. This phenomenon is the same for unicellular bacteria as well as for each of the trillions of cells found in the human body. Unlike prokaryotic cells, which have quick cell division to maintain homeostasis, eukaryotic cells rely on gene expression regulation to either increase quantities of one particular protein or a variation of proteins. It was previously thought that humans have hundreds of thousands of genes that express all of the proteins in our bodies. As it turns out, only 20,000 to 25,000 genes are coded in the genome (Human Genome Project). How is it that only 20,000 genes can make up all of the proteins that we need for an entire body’s survival? This is where the mechanisms of gene expression regulation give cells and organisms their diversity.
According to the central dogma of cell biology, while in the nucleus, the double-stranded DNA is first transcribed into RNA. This newly made strand of RNA then undergoes translation where it is made into a protein. At both of these steps there is an opportunity for gene expression regulation to occur to continue diversifying the composition of the cell. Gene expression regulation occurs even in the earliest stages of DNA replication (Figure 1). Cells have the capability of changing their chromatin structure using a remodeling complex, which condenses and decondenses itself. Decondensing allows for areas of DNA to be transcribed by polymerase (Alberts et al., p. 279). Another method of gene expression regulation at the structural level occurs through chemical modifications to the chromatin. Methylation and phosphorylation of the chromatin not only induce transient changes by attracting different proteins to those regions, but they also elicit permanent changes that then contribute to epigenetic inheritance that passes along these modifications (Alberts et al., p. 192).
Transcriptional control is the next process wherein gene expression regulation can occur. Transcriptional regulators can come in various forms, but all function to alter the rate of transcription from DNA to RNA by switching genes on or off (Alberts et al., p. 278). Once the precursor-messenger RNA (pre-mRNA) has been successfully transcribed, it is processed into mature mRNA where it can be even further regulated throughout RNA processing and throughout translation into protein.
There are many different ways for genes to be regulated during the processing of pre-mRNA to mRNA. Most mRNAs are capped at their 5’ end in order to stabilize the transcript and to guide the mRNA to the ribosome to begin translation (Gerstel et al., 1992; Alberts et al., p. 290). Another means of post-transcriptional regulation that gives stability to mRNAs and assists in translation is the process of polyadenylation, which is the addition of the nucleotide adenosine to the 3’ end of the mRNA (Alberts et al., p. 241). Two other post-transcriptional processes that regulate gene expression are alternative pre-mRNA splicing and RNA interference through microRNAs (miRNAs), which are the focus of this study.
Figure 1. The six steps of gene regulation shown in this figure are just a few examples of the many different ways genes can be regulated. While still in the nucleus, genes undergo regulation before and after transcription occurs. In genomic regulation, as seen in step 1, chromatin can condense or decondense to allow different genes to be transcribed. More regulation occurs through DNA modifications as seen in step 2. At this step, acetylation and methylation of the DNA can cause temporary or more permanent changes in gene expression. Once the strands of DNA have been transcribed by RNA polymerase II into pre-mRNA, capping of the 5’ end and alternative splicing of the pre-mRNA transcripts can occur as seen in step 3 of the post-transcriptional modifications. Boxes represent exons and lines represent introns that are spliced out via the spliceosome. Also occurring in step 3, is polyadenylation of the mRNA transcript to further ensure stability. The polyadenylated strand of mRNA is then transported out of the nucleus where it is translated into a protein. Gene expression regulation can also occur prior to translation through the formations of stem-loops on the mRNA transcripts. These stem-loops can cause the mRNA to be even more stable once out of the nucleus, and thus constitutes step 5 in the regulation. Further regulation can be seen through post-translation modifications as seen at step 6. Adapted from Figure 8-3 in Alberts, B., Bray, D., Hopkin, K., Johnson, A., Lewis, J., Raff, M., … Walter, P. 2010. Essential cell biology. New York, NY: Garland Science, Taylor & Francis Group.
Alternative pre-mRNA splicing
The RNA transcript that is transcribed from a gene contains non-coding sequences (known as introns) and protein-coding sequences (known as exons). After transcription, the introns must be removed and the exons must be ligated together to produce the mRNA that codes for a specific protein; this process is called pre-mRNA splicing. Non-coding regions are spliced out of the transcript by a large macromolecular complex known as the spliceosome.
Alternative splicing is one process of gene expression regulation where multiple mRNA transcripts can be made from one single pre-mRNA, ultimately leading to different protein isoforms. The alternative splicing process is crucial for gene expression regulation and cellular diversity because over 95% of all mRNA transcripts are alternatively spliced (Figure 2A; Pan, Shai, Lee, Frey, & Blencowe, 2008; Wang, Z. et al.,2008).
A standard human gene is comprised of approximately eight exons that are on average a couple of hundred nucleotides in length. An intron is between 3,000 and 5,000 nucleotides, making up approximately 90% of the standard gene (Tazi, Bakkour, & Stamm, 2009). The non-coding regions of the genome, such as introns, were—until recently—considered ‘junk’ that did not contribute to cellular processes. However, it is now clear that many important regulatory sequences and non-coding functional RNAs are found in these non-coding regions.
Regulatory sequences play an important role in recruiting the spliceosome to introns and exons and signaling where splicing should occur. There are four basic types of RNA binding elements that can be found in both exons and introns of a pre-mRNA transcript: exonic splicing enhancers (ESEs), exonic splicing silencers (ESSs), intronic splicing enhancers (ISEs), and intronic splicing silencers (ISSs) (Figure 2B). Enhancers function by promoting the inclusion of the exon that they are either located in or neighbor and are further regulated by RNA binding proteins (Wang & Burge, 2008).
A class of RNA binding proteins called serine/arginine-rich proteins (SR proteins) generally act to promote splicing. Individual SR proteins recognize and bind to specific ESE sequences and recruit spliceosomal machinery such as small nuclear ribonucleoproteins (snRNPs) to a nearby splice site (Bush & Hertel, 2012). Silencers function by inhibiting the inclusion of exons through recognition by heterogeneous nuclear ribonucleoproteins (hnRNPs) (Wang & Burge, 2008; Bush & Hertel, 2012). Splicing regulatory elements (SREs) provide extremely useful information during the process of alternative splicing as to which exons should be included or skipped in the transcripts, and regulation of these SREs provide another layer of gene expression regulation by modulating alternative pre-mRNA splicing.
Alternative splicing can regulate the levels of mRNA expression in many different ways. For example, alternative splicing can alter the function of a protein by changing the inclusion or skipping of functional or structural protein domains or by decreasing the stability of the mRNA transcripts (Stamm et al., 2005). These functions of alternative splicing can lead to a complete loss of function for some proteins, a gain of a new function, or give it an entirely new function in the cell (Stamm et al., 2005; Kelemen et al., 2012).
Figure 2. Overview of alternative pre-mRNA splicing and regulating mechanisms. A) During pre-mRNA splicing the spliceosome selects some exons and skips others to produce different forms of the pre-mRNA transcript. The removal of some exons and not others allows the same genetic material to produce different proteins. Part A shows that two different protein isoforms can be produced by either including or skipping the blue exon in the mRNA transcript. B) There are many regulatory sequences within pre-mRNA transcripts that direct the spliceosome during splicing. The orange boxes represent splicing enhancers and the red boxes represent suppressors. Enhancers and suppressors are found in both exons and introns and together they promote the inclusion or skipping of neighboring exons. MiRNAs, represented in green, can be found along the host transcript in exons, introns, and across the splice site. Is it possible for miRNAs to also regulate the splicing of their host gene?
Alternative splicing function and dysfunction
Although alternative pre-mRNA splicing may lead to new functions for proteins, more often it is the cause of cellular dysfunction—ultimately leading to disease. Some gene mutations are capable of disrupting alternative splice sites, resulting in a shift of functional protein isoforms, while others can disrupt or inactivate the regulatory machinery of alternative splicing (Faustino & Cooper, 2003). This is seen in the inactivation of the Wilms’ tumor suppressor gene (WT1) that is responsible for Frasier’s syndrome. Fraiser’s syndrome is characterized by impairment of kidney and gonad development. This inactivation of WT1 is due to a deletion of three amino acids, which causes an increase in a zinc finger protein isoform with non-functioning properties (Klamt et al., 1998).
Alternative splicing has also been implicated in the dysregulation of transcription factors, transmembrane proteins, and alterations in cell signaling, all of which have been associated with cancer (Venables, 2004). In a familial study done by Hoffman et al.(1998), five out of the nine subjects had an intronic single nucleotide polymorphism of G to A, and all of their relatives had some form of cancer. This single nucleotide polymorphism causes an insertion of only eleven nucleotides into the mRNA transcript of the BRCA1 gene. This mutation ultimately leads to a truncated protein, which is translated from the mutation (Hoffman, Hallam, Venne, Lyon, & Ward, 1998). The previous examples of dysfunction help to show how complex and precise the mechanism must be in order to produce a normally functioning protein. Alternative splicing is a ubiquitous process, so any mutations that occur during the process of splicing can be disastrous to many different parts of the body. This is especially the case when dysfunction of alternative splicing arises in the nervous system, where it is most abundant (Licatalosi & Darnell, 2006)
Neuronal pre-mRNA splicing function and dysfunction
Alternative pre-mRNA splicing is highly abundant in the brain and plays an important role in neuronal development and regulation of mature neurons (Yeo, Holste, Kreiman, & Burge, 2004; Li, Lee, & Black, 2007). Alternative splicing is known to regulate processes such as neuronal migration, synaptogenesis, and postsynaptic responses (Ullrich, Ushkaryov, & Sudhof, 1995; Li et al., 2007; Norris & Calarco, 2012). During synaptogenesis, over one thousand transcripts for neurexin have been found; demonstrating how prevalent alternative splicing is in neuronal tissue (Norris & Calarco, 2012). Spatio-temporal gene regulation has been shown to be very important in the development of the nervous system and throughout the body and helps to explain how tissues can express splicing patterning that is specific to that tissue and developmental stage (West & Greenberg, 2011). Although alternative splicing plays many important roles in the development of the nervous system, many neurological diseases have been associated with dysfunctions in alternative splicing. Spinal muscular atrophy (SMA) is characterized by the death of motor neurons exclusively in the anterior horns of the spinal cord. In 1995, Lefebreve et al. found that SMA is caused by a deletion in the survival of motor neuron 1 (SMN1) protein. Humans have a homologous gene, SMN2, which has a single nucleotide polymorphism of a C to a T, which disrupts the splicing of the protein. The SMN protein is extremely important for many processes of the cell because SMN associates with a protein complex that assembles snRNPs that are essential for pre-mRNA splicing (Liu, Fischer, Wang, & Dreyfuss, 1997). SMA is just one example of how important proper alternative splicing is to the function of proteins and cellular processes. Understanding what causes the disarray that arises from mutation in alternative splicing will help in developing new therapies and strategies for correcting aberrant splicing.
MicroRNA processing
The second post-transcriptional process focused in this study is microRNA biogenesis. The first miRNA was reported in Caenorhabditis elegans in 1993 with the discovery of lin-4, though it was not until 2000 that the second miRNA, let-7, was found in other species including mammals (Lee, Feinbaum, & Ambros, 1993; Reinhart et al., 2000; Pasquinelliet al., 2000). In 2010, the registry database of all miRNAs (miRBase) reported that it contained over 17,000 distinct miRNA sequences from 170 different species (Kozomara & Griffiths-Jones, 2010). MiRBase is an invaluable resource, providing an immense amount of information regarding where miRNAs are located and emphasizing their importance in the process of gene regulation across species.
MiRNAs are initially transcribed from genes and fold into stem-loops, approximately 80 nucleotides in length, and additionally are processed into their mature transcript length of 20-25 nucleotides (Shomron & Levy, 2009). RNA polymerase II transcribes miRNA genes into primary transcripts (pri-miRNAs) that can then be excised by the Microprocessor complex into pre-miRNAs (Lee et al., 2004). The Microprocessor complex is composed of two primary proteins, DiGeorge syndrome critical region gene 8 (DGCR8; or Pasha in Drosophila melanogaster and C. elegans), and Drosha (Lee et al., 2003; Han et al., 2004; Han et al., 2006). After excision by the Microprocessor complex, the pre-miRNA transcript is cleaved into an approximately 70-nucleotide long pre-miRNA (Denli, Tops, Plasterk, Ketting, & Hannon,2004). The pre-miRNAs are exported out of the nucleus by the nuclear export factor protein, exportin 5 (XPO5) where it is then processed into a miRNA duplex by the protein Dicer (Yi, Qin, Kreiman, & Burge,2003; Bohnsack, Czaplinkski, & Gorlich,2004; Lund, Guttinger, Calado, Dahlberg, & Kutay,2004; Bernstein, Caudy, Hammond, & Hannon,2001). Dicer is also involved in processing the mature miRNA into the RNA-induced silencing complex (RISC) where it forms a complex with Argonaute (AGO) 1-4 and other RNA-bindings proteins to carry out the mRNA silencing function (Ketting et al., 2001; Kim, 2005a).
MiRNAs can be located in various places in the genome: hosted exonically, intronically, and spanning a splice-site, but are primarily found in non-coding regions of the genome (intergenic regions) (Figure 3A; Kim, 2005b). Approximately 10% of the miRNAs found in coding regions of the genome are located within exons; the remaining 40% of miRNAs are located in introns. Some miRNAs comprise the entire length of small introns; these miRNAs are called mirtrons. Rather than undergoing cleavage by the Microprocessor, the mirtrons are spliced out by the spliceosome and then processed into mature miRNAs via the canonical pathway (Lai, 2003; Bartel, 2004).
Gene expression regulation, through miRNA processing, functions through a process called gene silencing. Regulation at the translational level occurs through three different processes: deadenylating the 3’ end of the mRNA transcript so that it becomes unstable, degrading the mRNA transcript so it cannot be translated into a protein, or inhibiting ribosomal translation of the mRNA so that a protein is not translated (Figure 3A; Fazi & Nervi, 2008). All three miRNA silencing mechanisms are ubiquitously found, and ultimately affect the protein that is translated from the mRNA. MiRNAs target mRNA transcripts by binding complementarily to their 3’ ends. Each individual miRNA can have hundreds of potential mRNA targets, and each target mRNA can be regulated by multiple miRNA making these two processes even more diverse. MiRNAs have many implications in neuronal and non-neuronal diseases, as discussed in the next section.
MicroRNA function and dysfunction
Regulation of miRNA abundance is important for regulating many biological functions such as cell differentiation, stem cell proliferation, and even specific regulations involved in aging (Kim, 2005a; Erson & Petty, 2008; Hackl et al., 2010). We find in many cases that the expressions of miRNAs are significantly different between normal and diseased tissue deeming miRNA expression a potential biomarker for many diseases. In patients with cancer, miRNAs function as tumor suppressors through regulation of apoptosis and oncogenes through inhibition of tumor suppressor genes (Erson & Petty, 2009). In 2002, the first miRNAs involved in cancer were demonstrated in human patients with Chronic Lymphocytic Leukemia (CLL) (Calin et al., 2002).
Figure 3. Processing of miRNA, how it functions in the RISC complex, and its involvement in disease. A) Pri-miRNAs exist in the transcript of pre-mRNA in the form of a hairloop structure about 70 nucleotides in length. The pri-miRNA is cleaved out of the transcript by the Microprocessor comprised of Drosha and DGCR8 to form pre-miRNA. Exportin5 is responsible for transporting the pre-miRNA out of the nucleus and into the cytoplasm where it is further shortened. While in the cytoplasm, Dicer further crops the pre-miRNA into the mature miRNA duplex. One strand of this duplex is then incorporated into the RISC complex with the help of Ago proteins. Once incorporated into RISC, the complex can now regulate mRNA via degradation of the mRNA transcript, inhibition of mRNA translation, or deadenylation of the mRNA. Figure adapted from Cullen, 2005 and Fazi & Nervi, 2008. B) Part B shows examples of diseases, both neuronal and non-neuronal, that miRNAs are implicated in. All models have been shown in human patients with the exception of the polycystic kidney disease shown in a mouse model.
Sixty-eight percent of CLL patients had deletions in a small region of their genomes, which coded for the genes miR-15 and miR-16 (Calin et al., 2002). Many more miRNA levels have been found to be dysregulated in patients with cancers such as colorectal cancer, lung tumor tissues, breast tumors, other lymphomas, and in brain cancer (Lu et al., 2005; Zhang, Pan, Cobb, & Anderson,2007).
Neuronal microRNA function and dysfunction
Spatio-temporal patterning of miRNAs is also highly evident in brain tissue as nearly 50% of all miRNAs are expressed in neural tissue and play an important role in the structuring of the brain (Krichevsky, King, Donahue Khrapko, & Koski,2003; Shao et al., 2010). A study using Dicer knockout zebrafish showed that gastrulation, neural development, and other non-neural developmental processes were severely affected when devoid of miRNAs; brain morophogenesis was rescued, however, when miR-430 was reintroduced to the brain through injections (Giraldez et al., 2005). MiRNAs have also been implicated in the functioning of the circadian clock. Cheng et al.(2007) showed that two brain-specific miRNAs, miR-219 and miR-132, played critical roles in regulating different proteins in the suprachiasmatic nucleus (SCN) an area of the brain associated with circadian rhythms. After knockdown of miR-219, the length of the circadian cycle increased by over 0.3 hours (Cheng et al., 2007).
Though miRNAs play important roles in the development of different processes, if expressions of miRNAs become dysregulated many diseases and disorders occur. In rats that had access to self-administered cocaine, miR-212 was upregulated compared to control rats, suggesting that it plays a major role in the receptor binding in the reward circuitry (Hollander et al., 2010). MiRNA dysregulation is also seen in many neurological diseases such as Tourette’s syndrome, Alzheimer’s disease, Huntington’s disease, and Parkinson’s disease (Kuss & Chen, 2008; Christensen & Schratt, 2009). The first link between miRNAs and disease in neurons came from cases of patients with Fragile X syndrome (Kuss & Chen, 2008). Fragile X syndrome is caused by the loss of the Fragile X Mental Retardation Protein (FMRP), which associates with AGO proteins found in the RISC complex of the miRNA regulation pathway (Jin et al., 2004). Dicer knockouts are used to study what happens to neuronal cells when there is a complete loss of miRNA. These studies report loss of different cellular proteins and function leading to cellular death (Schaefer et al., 2007). Understanding the regulation of miRNAs in these varying neurological diseases allows for potential therapeutic options for different disorders and diseases by either restoring the lost miRNAs to the system or suppressing those that are overexpressed.
My Project
Gap of knowledge and purpose
Until recently, much of the post-transcriptional regulation focus has been on how miRNAs can regulate gene expression at the level of mRNA to protein, and little research has been done on how miRNA biogenesis and alternative pre-mRNA splicing coexist.
The purpose of this study was to better understand how two different RNA transcripts (miRNA and mRNA) can originate from the same pre-mRNA, and if there is any co-regulation between the processes of splicing and miRNA biogenesis. We looked at the splicing of host gene eIF4H and its intronically hosted miRNA, miR-590, to examine the effects to each process when the other has been altered. Furthermore, we looked to see if the Microprocessor has a role outside of the miRNA biogenesis pathway.
Hypothesis
We hypothesized that the two processes, host gene alternative pre-mRNA splicing and miRNA biogenesis are co-regulated, and that miRNA biogenesis impacts the inclusion of alternative exons in the final mRNA transcript.
Aim #1: Assess co-regulation between pre-mRNA splicing and miRNA biogenesis
In this study, we examined alternative splicing of four different genes that have a miRNA within an intron that flanks an alternatively spliced exon. To test whether there was co-regulation between splicing of the so-called host gene mRNA and miRNA processing, we manipulated splicing and analyzed the effects on miRNA biogenesis, and then conversely manipulated miRNA biogenesis and analyzed the effects on pre-mRNA splicing. We found that manipulations to splicing had widespread effects on miRNA abundance, and manipulations of miRNA biogenesis affected the proportion of full-length to skipped transcript in some cases. These results support our hypothesis that these two processes influence each other and may be co-regulated.
Aim #2: Determine the mechanism of co-regulation
To further assess the mechanism behind the co-regulation that was seen in the findings of aim #1, we looked specifically at the alternative splicing of host gene eIF4H and the production of its miRNA, miR-590, which is located in the intron downstream of alternatively spliced exon 5. Alternative splicing of exon 5 gives rise to two eIF4H protein isoforms based on the inclusion or skipping of exon 5. In 2010, Wu et al. reported overexpression of full-length eIF4H in colorectal cancers. Furthermore, overexpression of the isoform of eIF4H, which includes exon 5 in the mRNA transcript, led to gastrointestinal tumors in mice, and suppression of the full-length isoform of eIF4H inhibited proliferation of colon cancer cells (Wu, Matushita, Matsubara, Nomura, & Tomonaga, 2010).
EIF4H is part of a family of eukaryotic initiation factors (eIFs) that play a role in translation regulation. The family of eIFs includes subclasses eIF1-4 and promotes translation by anchoring the mRNA transcript to the ribosomal complex (Figure 4A). The eIF4H protein was found to interact with the mRNA, stimulate the initiation of protein synthesis, and stabilize other eIF4s (Richter-Cook, Denber, Hensold, & Merrick,1998; Richter, Rogers, Hensold, & Merrick,1999). We chose to use eIF4H based on the two splicing variants that are formed during alternative splicing and because eIF4H hosts miR-590 in the fifth intron of its gene (Figure 4B). We overexpressed and knocked down components of the Microprocessor to examine whether changes to miRNA biogenesis had effects on splicing of host gene eIF4H. Additionally, we knocked down splicing proteins to alter the splicing of eIF4H,and analyzed the effects on miR-590 production. We found that the altering expression of miRNA biogenesis proteins had no significant effect on the splicing of eIF4H. In contrast, knockdown of specific splicing proteins increased exon 5 splicing and also increased miR-590 abundance. These findings provided evidence that splicing or splicing proteins are involved in miRNA biogenesis, suggesting that the two mechanisms may be co-regulated.
Figure 4. Function of eIF4H in cells and its dysfunction in gastrointestinal cancer. A) eIF4H is part of a family of eukaryotic initiation factors (eIFs) that function in the translational phase. eIFs function specifically to anchor the mRNA transcript to the ribosome and promote translation. There are four subclasses of eIFs (eIF1, eIF2, eIF3, and eIF4). B) Of the subclass eIF4, we are interested in looking at the splicing of eIF4H. In gastrointestinal cancer, two different isoforms of eIF4H are found. In normal tissue, exon 5 of eIF4H is skipped in the mature mRNA transcript. In cancer tissue, however, an isoform with exon 5 included is upregulated. MiR-590 is hosted in intron 5 of eIF4H. This provokes the question: can miRNA regulate host gene alternative pre-mRNA splicing?
Figure 5. My project thesis and aims. We hypothesized that host gene alternative pre-mRNA splicing and miRNA biogenesis are co-regulated. A) Aim 1 attempts to assess the co-regulation of these two processes through the manipulation of either pre-mRNA splicing to see the effects on miRNA biogenesis, or the manipulation of the Microprocessor to see the effects on alternative pre-mRNA splicing. B) Aim 2 attempts to further understand the mechanism behind the co-regulation of these two processes by using host gene eIF4H and its intronic miR-590.
Results
Experimental design for aim #1
We hypothesized that manipulations of host gene splicing would have an effect on miRNA biogenesis, and manipulations to the Microprocessor would have an effect on the splicing of four host genes: eIF4H, SLIT2, EGFL7, and PPIL2, which houses miR-590, miR-130b, miR-126, and miR-218 respectively. Two experiments were used to examine this possibility: one utilized overexpression of a U1 snRNP decoy that sequesters a core component of the spliceosome to decrease splicing efficiency, and the other overexpresses a dominant-negative form of the Microprocessor component, Drosha. Since the U1 snRNP is the main recruiter of the other components of the spliceosome, we expected to see an increase of exon skipping of both miRNA host genes and non-host genes. We further hypothesized that increasing exon skipping would also increase the amount of miRNA produced.
The second experiment introduced a Transdominant Negative (TN) Drosha into the cells. TN Drosha can still interact with DGCR8 and bind to pri-mRNA structures, but does not cleave them into pre-miRNA (Heo et al., 2008). Therefore, introducing TN Drosha to the cell would decrease the total amount of miRNA produced. We expected that introducing TN Drosha would decrease overall miRNA production. Furthermore, we hypothesized that this would increase the full-length mRNA transcript abundances of only mRNAs that were host to an intronic miRNA, and would not alter splicing of non-host genes.
Expression of a U1 snRNP decoy alters host gene splicing and miRNA abundance
To determine whether co-regulation between alternative pre-mRNA splicing and miRNA biogenesis exists, we first manipulated splicing to then see if there were any alterations to miRNA abundance. HEK-293T cells were treated with either the U1 decoy plasmid (FL) or a control plasmid (2C). The control plasmid had a point mutation in the U1 snRNP decoy sequence that disrupts binding of the U1 snRNP. Forty-eight hours after transfection, RNA was analyzed for abundance of miRNA. The proportions of full-length to skipped mRNA transcripts in four different host genes containing a miRNA were also analyzed after transfection.
SMN was chosen as a positive control because it does not house a miRNA in its transcript; however, SMN still requires U1 for splicing, and alternative splicing of SMN has been modified following U1 decoy . Both forms of SMN (SMN1 and SMN2) showed significant decreases in the proportion of full-length transcript to Δexon 7 skipped transcript in the U1 decoy treatment compared to the control treatment (p<0.05; Figure 6A). These results confirmed that the U1 decoy system functioned as expected.
The eIF4H host gene also showed a significant decrease in the proportion of full-length to Δexon 5 skipped transcript in the U1 decoy treatment compared to the control treatment (p<0.05; Figure 6B). Host gene to miR-130b, PPIL2, showed a decrease in the proportion of full-length to Δexon 2 skipped transcripts, but was not statistically significant (p>0.05). The decrease seen in the full-length to Δexon 7 skipped transcript in EGFL7, host to miR-126, and full-length to Δexon 15 skipped transcript of SLIT2, host gene to miR-218, were also not statistically significant (Figure 6C; p>0.05).
To analyze the effect of the U1 decoy expression and splicing alterations on the expression of miRNAs, we hybridized the RNA isolated from U1 decoy expressing cells and control cells to a miRNA microarray. We found that some miRNA were upregulated, while others downregulated, in the U1 decoy expressing samples (Figure 7A). The miRNAs of interest are color coded and mapped to their specific locations in the microarray graph: miR-590 is colored in red, miR-130b is colored in purple, miR-126 is colored in green, and miR-218 is colored in orange. The results of the microarray in the U1 decoy experiments by stem-loop RT-PCR showed a trend towards lower abundance of miR-590 expressed in the U1 decoy samples compared to the control treatment (p>0.05; Figure 7B). Similarly, miR-130b and miR-126 decreased in U1 decoy samples in the microarray experiment and this trend was validated by stem-loop RT-PCR for miR-130b, but not miR-126 (p>0.05; Fig. 7B). Together, these results, though preliminary, may suggest that a decrease in exon splicing (caused by U1 decoy expression) correlates with a decrease in host miRNA expression. This observation may indicate cooperation between splicing and miRNA processing.
Figure 6. Sequestering the U1 snRNP increases exon skipping in pre-mRNAs. RT-PCR samples ran through gel electrophoresis to analyze mRNA transcript abundance. A representative gel image is shown for each set of samples along with the average of the quantifications for all three replications. A) Control gene SMN, which does not house a miRNA, showed that U1 decoy successfully increases the amount of exon skipping as seen in both SMN1 and SMN2 (p<0.01; p<0.05). B&C) All four miRNA host genes (eIF4H, host to miR-590, PPIL2, host to miR-130b, EGFL7, host to miR-126, and SLIT2, host to miR-218) showed a decrease in the amount of full-length mRNA transcript and conversely an increase in the amount of exon skipping. B) The decrease seen in the proportion of full-length to skipped transcript in eIF4H was statistically significant (p<0.05).
Figure 7. MiRNA abundance is affected by sequestration of U1 snRNP. RT-PCR samples ran through gel electrophoresis to analyze mature miRNA abundance. A representative gel image is shown for each set of samples along with the average of the quantifications for all three replications. A) A microarray used to quantitate levels of miRNA, in the presence of the U1 decoy, showed large-scale changes in miRNA abundance in HEK-293T cells. MiRNA levels in U1 decoy condition were normalized to miRNA levels in control condition. MiRNAs of interest (miR-590, hosted in eIF4H, miR-130b, hosted in PPIL2, miR-126, hosted in EGFL7, and miR-218, hosted in SLIT2) are mapped on to the graph of the entire microarray. MiR-590 is colored in red, both species of miR-130b are colored in purple, miR-126 is colored in green, and miR-218 is colored in orange. B) Abundance of miR-590 was specifically examined in the U1 decoy experiment, and was found to not be significant different from levels found in the control conditions (p>0.05). miR-130b, miR-126, miR-218 levels were also examined, and found to decrease in the case of miR-130b and miR-218, and increase in the case of miR-126, however, none of these trends were statistically significant (p>0.05).
TN Drosha expression alters miRNA abundance and host gene splicing
We next wanted to examine whether alterations to miRNA biogenesis had any effect on host gene splicing. HEK-293T cells were treated with either the TN Drosha plasmid or a mock control treatment where no plasmid was introduced. The abundance of miRNA as well as the proportion of full-length to exon skipping of the mRNA transcript of the four host genes containing a miRNA as well as negative control gene, SMN, was determined.
The abundance of miR-590, miR-130b, miR-126, miR-218 was normalized to snoRNA65. All four miRNAs showed a decrease in their abundance normalized to snoRNA65, and in the case of miR-590 and miR-126 statistically significant decreases were observed (p<0.05; Figure 8).
Splicing of the negative controls SMN1 and SMN2 transcripts showed no statistically significant decrease in the proportion of full-length to exon 7 skipped transcript (p>0.05; Figure 9A). The proportion of full-length to exon 5 skipped transcript in host gene eIF4H, however, was shown to statistically increase in the TN Drosha treatment compared to the mock control treatment (p<0.05; Figure 9B). The decrease in full-length to exon 2 skipping as seen in the transcript of the PPIL2 host gene, the decrease in full-length to exon 2 skipping as seen in the transcript of the EGFL7 host gene, and the decrease seen in full-length to exon 15 skipping as seen in the transcript of the SLIT2 host gene were not statistically significant (p>0.05; Figure 9C). These results indicate that decreasing Drosha activity, by expressing the dominant-negative TN Drosha, not only lowers miRNA abundance, as expected, but also changes alternative splicing levels. The decrease in host miRNA does not correlate consistently with an increase or decrease in alternative exon splicing, perhaps suggesting that the interplay between the miRNA processing and splicing may be context dependent.
Figure 8. TN Drosha decreases miRNA abundance. RT-PCR samples ran through gel electrophoresis to analyze miRNA abundance. A representative gel image is shown for each set of samples along with the average of the quantifications for all ten replications. The use of dominant-negative Drosha (TN Drosha) was shown to generally decrease the amount of miRNA that is produced in HEK-293T cells. The decreases seen in miR-590 and miR-126 were statistically significant (p<0.01) relative to the mock control; however, the differences seen in miR-130b and miR-218 were not statistically significant (p>0.05).
Figure 9. TN Drosha alters host gene splicing, but not the splicing of genes not hosting miRNAs. RT-PCR samples ran through gel electrophoresis to analyze mRNA transcript abundance. A representative gel image is shown for each set of samples along with the average of the quantifications for all three replications. A) Control gene SMN, which does not house a miRNA, does not show a significant difference in the proportion of full-length to skipped transcripts in the presence of TN Drosha. B) The splicing of eIF4H, host to miR-590, showed a significant increase in the proportion of full-length to skipped transcript in the presence of TN Drosha (p<0.05). C) PPIL2, host to miR-130b, EGFL7, host to miR-126, and SLIT2, host to miR-218, all showed slight decreases in the proportion of full-length to skipped transcripts, but none of those differences were statistically significant (p>0.05).
Experimental design for aim #2
We then focused our next experiments on the splicing of host gene eIF4H and miR-590 production to determine the Microprocessor’s role in alternative splicing. We hypothesized that alterations of different components of the Microprocessor would affect alternative pre-mRNA splicing. In order to test this hypothesis, we first used RNAi via small interfering RNAs (siRNAs) to knockdown the amount of DGCR8 in the cell. By knocking down DGCR8, we should see an overall decrease in the amount of miR-590 produced in the cell. We also hypothesized that there would be an increase in the proportion of full-length to skipped transcripts.
The second experiment introduced a dominant-negative form of Dicer (TN Dicer) into cells to determine if alterations to downstream miRNA processing components had any effect on splicing of eIF4H. This experiment served as a negative control because Dicer’s role in the processing of miRNAs occurs after excision of the pri-miRNA from the mRNA transcript. Therefore, we expect to see a decrease in the abundance of miR-590 and no changes in the splicing of host genes and non-host genes.
In our third experiment, we sought to increase the cellular abundance of miRNA processing machinery by overexpressing the amount of Drosha and Dicer in HEK-293T cells. We expected that overexpression of the components would lead to increases in miR-590 production, but only overexpression of Drosha would result in an increase in the abundance of the skipped transcript of eIF4H. In this experiment, overexpression of Dicer served again as a negative control to verify that any alterations seen in splicing could be attributed to miRNA processing and not miRNA targeting.
Finally, we analyzed the effects on miRNA biogenesis when we manipulated splicing by knockdown of various splicing factors to determine if any other regulators of eI4FH splicing also affected miR-590 production.
Depletion of DGCR8 does not affect alternative splicing of eIF4H
After our results confirmed that TN Drosha had an effect on host gene splicing, we next looked at the other component of the Microprocessor, DGCR8, to see if it also affected splicing of the host gene. HeLa cells were treated with DGCR8-specific siRNAs or a mock control and analyzed for both the abundance of miR-590 and the proportion of full-length to exon 5 skipping transcript of eIF4H.
We used RT-PCR as well as a Western blot analysis of protein abundance to verify the decrease of DGCR8 in cells. Using RT-PCR we found that DCGR8 transcript levels in the RNAi samples were 43% of the abundance quantitated in the mock control samples. GAPDH was used as a loading control and levels of DGCR8 transcript were normalized to the abundance of GAPDH. A Western blot was used to analyze the protein expression of DGCR8. β-actin was used as a loading control and levels of DGCR8 protein were normalized to that of β-actin. DGCR8 levels were reduced to 54% of the abundance found in the mock control samples (Havens et al., 2012).
Analysis of eIF4H’s alternative splicing showed no significant difference between the proportion of full-length to skipped transcript in the control compared to siDGCR8 samples (p>0.05; Figure 10A). SnoRN65 was used as a loading control and abundance of miR-590 were normalized to levels of snoRNA65 in each sample. The abundance of
Figure 10. mRNA alternative splicing is not altered by DGCR8 RNAi. RT-PCR samples ran through gel electrophoresis to analyze mRNA transcript and miRNA abundance. A representative gel image is shown for each set of samples along with the average of the quantifications for all five replications. A) Splicing of eIF4H was slightly altered, but not significantly (p>0.05). The proportion of full-length to skipped isoform slightly increased in the siDGCR8 samples. B) The abundance of miR-590 in siDGCR8 was significantly decreased compared to mock control samples (p<0.05). The arrow denotes the miR-590 band, and the band below is PCR debris. SnoRNA65 was used as a loading control for the RT-PCR samples of miRNA.
miR-590 significantly decreased in siDGCR8 samples compared to the mock control samples (p<0.05; Figure 10B).
Dominant-negative Dicer expression does not alter eIF4H alternative splicing
To determine whether the alterations to splicing seen in the TN Drosha experiments were due to targeting of the host mRNA, we used a dominant-negative form of Dicer. Since Dicer has downstream functions in miRNA processing, we did not expect to see a change in the splicing of eIF4H in TN Dicer samples. HEK-293T cells were treated with either the TN Dicer plasmid or a mock control treatment, where no plasmid was introduced. Both abundance of miR-590 and the proportion of full-length to exon 5 skipping of the eIF4H were analyzed. The splicing of the negative control genes, SMN1 and SMN2, were also analyzed in both mock control and TN Dicer samples.
Splicing of the negative controls SMN1 and SMN2 showed no statistically significant decrease in the proportion of full-length to Δexon 7 skipped transcript (p>0.05; Figure 11A). The proportion of full-length to Δexon 5 skipped transcript in host gene eIF4H was shown to have no statistically significant changes in the TN Dicer treatment compared to the mock control treatment (p>0.05; Figure 11B). The abundance of miR-590 was normalized to snoRNA65, and was shown to decrease in TN Dicer samples compared to mock control in n=1 (Figure 11C). As expected, our results demonstrate the TN Dicer does not affect host gene splicing.
Figure 11. TN Dicer shows no changes in splicing of eIF4H. RT-PCR samples ran through gel electrophoresis to analyze mRNA transcript and miRNA abundance. A representative gel image is shown for each set of mRNA samples along with the average of the quantifications for all three replications. A) Control gene SMN1 and SMN2, which does not house a miRNA, does not show a significant difference in the proportion of full-length to skipped transcripts in the presence of TN Dicer (p>0.05). B) The splicing of eIF4H showed no significant change in the proportion of full-length to skipped transcript in the presence of TN Dicer (p>0.05). C) MiR-590 abundance was normalized to snoRNA65. TN Dicer decreased compared to mock control in n=1.
Overexpression of microRNA processing proteins alter splicing of eIF4H
We next overexpressed proteins involved in miRNA processing to further study the Microprocessor’s role in splicing co-regulation. HeLa cells were transfected with plasmids expressing GFP control, Drosha, and Dicer to analyze overexpression of these transcripts, miR-590 production, and alternative splicing of eIF4H.
We used RT-PCR to analyze the transcripts for Drosha and Dicer overexpression in the samples. GAPDH was used as a loading control and levels of transcript were normalized to abundance of GAPDH for each sample. There was a significant increase in both Drosha and Dicer transcripts compared to GFP control samples (p<0.05; Figure 12A). Alternative splicing of eIF4H was analyzed and compared to GFP controls, which showed a significant increase in the proportion of full-length to exon 5 skipped transcript (p<0.05; Figure 12B). No significant difference was seen in Dicer overexpression samples compared to GFP control samples (p>0.05; Figure 12B). SnoRNA65 was used as a loading control and abundance of miR-590 was normalized to levels of snoRNA65 in each sample. When compared to the GFP control, no significant difference was seen in miR-590 abundance compared (p>0.05; Figure 12C). These results provided evidence that overexpression of Drosha actually increases the proportion of full-length to skipped transcript, which did not support our original hypothesis. Overexpression of Dicer showed no significant effect on the proportion of full-length to skipped transcript.
Figure 12. Overexpression of Drosha and Dicer alters host gene splicing, but not miR-590 abundance. RT-PCR samples ran through gel electrophoresis to analyze mRNA transcript and miRNA abundance. A representative gel image is shown for each set of samples along with the average of the quantifications for all three replications. A) RT-PCR was used to verify the overexpression of Drosha and Dicer. GAPDH was used as a loading control and levels of Drosha and Dicer were normalized to the amount of GAPDH in each sample. Flag GFP treated cells were used as a control to compare the normalized amounts of Drosha and Dicer transcript. Drosha and Dicer transcripts were significantly increased (p<0.01). B) Alternative pre-mRNA splicing of eIF4H was significantly increased in Drosha samples when compared to Flag GFP control (p<0.05). No significant difference in splicing was seen in Dicer samples compared to control (p>0.05). C) MiR-590 abundance in both Drosha and Dicer samples increased, but were not significantly different from the Flag GFP control (p>0.05).
Depletion of SR protein class of splicing factors (SRSFs) affect alternative splicing
We then looked to see if there were any other regulators of eIF4H splicing that also had an effect on miR-590 production. First, we surveyed a panel of SR proteins that contained binding regions to eIF4H. HeLa cells were treated with SRSF-specific siRNAs to knockdown various SR proteins in the cells. Alternative splicing of the eIF4H transcript and miR-590 abundance were analyzed for each sample.
Western blot was used to verify that the SRSFs at the protein level had been sufficiently reduced in the samples. An overall decrease in protein abundance was seen for each siRNA treated sample when compared to the allstar control (data not shown).
A significant increase in the proportion of full-length to Dexon 5 skipped transcript was seen in SRp30b and SRp75 out of the 12 siSRSF samples compared to allstar control suggesting that SRp30b and SRp75 inhibit exon splicing (p<0.05; Figure 13B).
Depletion of additional regulatory splicing factors affect alternative splicing
Next, we looked at several hnRNPs as possible splicing regulators to see if they also had an effect on miR-590 production. HeLa cells were treated with splicing factor-specific siRNAs to knockdown various splicing factors in the cells. Western blot analysis was employed to verify that the siRNAs were working to knockdown the splicing factors and reduced the amount of protein produced. An overall decrease was seen for each siRNA treated sample compared to the allstar control (data not shown).
Figure 13. Knockdown of SR Splicing Factor (SRSF) proteins. RT-PCR samples were ran through gel electrophoresis to analyze mRNA transcript abundance. A representative gel image is shown for each set of samples along with the average of the quantifications for all three replications. Twelve different SR proteins were knocked down in a panel to test splicing of eIF4H. We founda significant increase in the amount of full-length to skipped transcript after knockdown of SRp30b and SRp75 (p<0.05).