Channeling epilepsy’s hyper-active potential
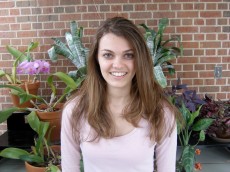
Victoria Egedus
Department of Biology
Lake Forest College,
Lake Forest, IL 60045
Download PDF
Abstract
Epilepsy is a brain disorder defined by a person having two or more unprovoked seizures. Most seizures are caused by hyper excitable neuronal activity and may affect a number of behaviors, including motor, sensory, cognitive or autonomic functions. A broad range of seizure types can occur, and many are linked to mutations in ion channels. This review focuses on four ion channel mutations directly related to major forms of epilepsy. Mutations in the voltage-gated sodium channel cause prolonged sodium influx, which lead to extended depolarization. Voltage-gated potassium channel mutations lead to a slow repolarization during the refractory period, and lead to repeated action potentials. Mutations in the ligand-gated nicotinic acetylcholine receptor cause increased sensitivity to acetylcholine activation and overexcite the thalamo-cortical loop. Mutations in the ionotropic GABAA receptor can accelerate decay of phasic GABA currents and decrease tonic, non-synaptic currents. More recent studies have also looked into the role of glial cells in epilepsy pathology, as they are responsible for the uptake of synaptic glutamate. Activated glia in epileptic loci may also exaggerate neuron excitability through an enhanced rate of glutamate release. While treatment for epilepsy includes medication, vagus nerve stimulation, surgery, and a specialized ketogenic diet, innovative new treatments are currently being developed, such as photoregulation of GABAA receptors.
Introduction
Epilepsy is a brain disorder characterized by repeated seizures. According to the Centers for Disease Control and Prevention, about two million Americans have epilepsy and one out of ten people will experience a seizure during their life. Medically documented for centuries, seizures were historically attributed to supernatural forces, or even witchcraft; but as Hippocrates argued in 400 B.C., seizures are as biological as any other brain disorder (Epilepsy website).
Seizures occur when there is a loss of balance in electrical activity in the brain. Individual neurons become overexcited and quickly solicit entire neuronal circuits in patterns of hyperexcitability, resulting in a seizure. An excess of glutamate, the most prevalent excitatory neurotransmitter, as well as underutilization of the inhibitory neurotransmitter GABA, are responsible for the observed phenotype.
Because the disorder is based in the brain, seizure types correlate to the location and area of abnormal brain activity. Experts classify seizures into four broad categories: generalized, partial, non-epileptic and status epilepticus (The Epilepsy Foundation website). Generalized seizures affect both hemispheres of the brain, and partial seizures are limited to one hemisphere. Non-epileptic seizures are often mistaken for epileptic seizures, but the behavioral changes linked to these seizures are not related to electrical brain activity and epilepsy medications have no effect on these episodes. Status epilepticus seizures are the most dangerous type of seizures, and last much longer than typical epileptic seizures. This type of seizure is unremitting and calls for serious medical attention. Symptoms of a seizure may involve disruptions of motor, sensory, cognitive or autonomic functions (reviewed in Steinlein, 2004), including severe body convulsions, loss of consciousness (known as absence), and loss of alertness. The cause of seizures can be a stroke, brain injury, infection, such as meningitis or AIDS, congenital birth defect, brain tumor, or use of certain medications such as antidepressants (PubMed Health website). However, most often seizures occur with no apparent cause.
Because seizures are so heterogeneous and a person may experience more than one type, “epilepsy syndromes” serve to classify subgroups of the disease based on a number of characteristics. These characteristics include seizure type, age of onset, factors that induce seizures, course of the disorder, response to treatment, area of the brain involved, and EEG recordings during and between seizures (Epilepsy website). There are more than fifty epilepsy syndromes, many of which pertain to children, including childhood absence epilepsy, juvenile myoclonic epilepsy, febrile seizures, Rasmussen syndrome, benign rolandic epilepsy, temporal lobe epilepsy, frontal lobe epilepsy, symptomatic generalized epilepsy, and reflex epilepsy.
Twenty years ago, epilepsy research was concentrated in employing animal models. Study animals were either predisposed to seizures, or drugs or electrical stimulation induced seizures. Animal modeling provides a way to study underlying neuronal mechanisms, identify genetic mutations, and search for anticonvulsant drugs. For example, animals such as epileptic dogs, tottering mice, rats, baboons, fowl and gerbils model distinct seizure types, and serve as great models for anticonvulsant drugs in humans (Loscher, 1984). Rodent models bred for seizure expression have been highly used to identify and locate genetic defects associated with seizures (Buchhalter, 1993). Use of chemical convulsants in animal models provided insight that GABA antagonists induce seizures, which are reversed by antiepileptic drugs, thereby supporting the GABAergic hypothesis (Biziere & Chambon, 1987). Chemical analysis also showed that animal models prone to epilepsy have altered transmission of neurotransmitters including serotonin, GABA and glutamate (Biziere & Chambon, 1987).
It wasn’t until 1995 that the first inherited epilepsy gene was identified in a group with autosomal dominant nocturnal frontal lobe epilepsy (ADNFLE). Since that time, idiopathic forms of epilepsy have been linked to over 25 mutant genes, constituting the main focus of epilepsy research. Many mutations are located in subunits of voltage-gated ion-channels, such as the sodium channel (SCN1A, SCN2A, SCN1B), potassium channel (KCNQ2, KCNQ3, KCNA1, KCNMA1, KCNJ11), calcium channel (CACNA1H, CACNA1A, CACNB4), chloride channel (CLCN2), and hyperpolarization-activated cyclic nucleotide-gated channel (HCN1, HCN1). Mutations have also been identified in ligand-gated ion channels, such as the nicotinic acetylcholine receptor (CHRNA4, CHRNB2, CHRNA2), and GABAA receptor (GABRG2, GABRA1, GABRD), as well as non-ion channel proteins (see Reid, Berkovic, & Petrou, 2009). The majority of the described mutations are in ion channel proteins and this review will focus on the mechanism of four ion channels involved in genetic cases of the disease. Additionally, the roles of glial cells in epilepsy pathology will be introduced. Refer to Figure 1 for an overall illustration regarding the cellular mechanisms discussed. Finally, there will be a brief overview of past, current and developing methods of epilepsy treatment.
Ligand-gated nicotinic acetylcholine receptor
As a ligand-gated ion channel, the nicotinic acetylcholine receptor (nAChR) is selectively bound by the neurotransmitter acetylcholine, which induces the channel to open. In the closed state, leucine residues in α helices of the M2 segment (which forms the channel wall) point toward the inside of the pore. Upon ligand binding, rotational changes move the leucine residues and open the pore. The receptor is permeable to sodium, potassium and calcium ions, and the overall effect of channel opening is net depolarization. While calcium influx may induce cellular changes such as neurotransmitter release or gene expression, the main focus in regards to the channel and epilepsy is that depolarization activates voltage-gated ion channels, thereby generating an action potential. The first mutation ever linked to idiopathic epilepsy was identified in nAChR (Steinlein et al., 1995). Scientists hypothesized that an alteration in nAChR function directly disturbs electrical signaling and thereby, leads to hyperexcitation.
The study by Steinlein et al. was conducted using DNA from 21 kin with autosomal dominant nocturnal frontal lobe epilepsy (ADNFLE), and identified a mutation at codon 248 of the α4 subunit (CHRNA4), where the highly conserved serine was replaced by phenylalanine (1995). ADNFLE is characterized by frequent, violent seizures during non-REM sleep, and usually begins in childhood (Scheffer et al., 1995). The physiological importance of the 248 serine residue is highlighted by its high conservation among vertebrate and invertebrate species (Steinlein et al., 1995), and site-directed mutation of serine 248 to valine or tyrosine inhibits sodium and potassium diffusion, while S248A alters the dissociation rate of channel blockers (Devillers-Thiery et al., 1993).
These data sparked the forthcoming understanding that idiopathic epilepsies are channelopathies and new hypotheses addressed the unique ADNFLE phenotype where seizures occur almost exclusively during sleep. Cholinergic neurons are directly involved in sleep and wakefulness in the thalamic and cortical systems (Steriade et al. 1993), so nAChR defects could provide a unique explanation for the observed phenotype. Therefore, it was postulated that the role of nAChR mutations related to epilepsy are most consequential in thalamocortical neurons.
While ADNFLE is a singular epilepsy syndrome that shows autosomal dominant inheritance, most idiopathic epilepsies are inherited in a complex manner. Nonetheless, autosomal dominant epilepsies provide a direct and reliable group for genetic inheritance studies, and this discovery marked the beginning of a new world in epilepsy research. It was now possible for epileptic models to be generated directly from a known epilepsy mutation.
Numerous studies have been conducted since that initial discovery, and many additional mutations in the α and β subunits (CHRNA4 and CHRNB2) have been reported. All mutations are located in the second or third transmembrane domain, suggesting that only mutations located directly within the channel pore wall can cause ADNFLE (reviewed in Steinlein, 2004). The emerging hypothesis was that the different α and β subunits must have a similar functional default that leads to epilepsy pathology.
Bertrand et al. took to characterize similarities and differences in the functioning of four different ADNFLE nAChR mutations in order to identify features in a common mechanism (2002). As an improvement to earlier nAChR studies, the study used cDNA amplified directly from patients with epilepsy, which was coexpressed with wildtype DNA in order to mimic heterozygous expression as in patients (Bertrand et al., 2002). Previous studies reported results with homozygous expression of the mutant subunit, which is not an ideal model for ADNFLE inheritance. By utilizing a Xenopus laevis oocyte model, the group showed that heterozygous expression of the α4 subunit S248F mutation displayed higher desensitization (meaning that the channel closes more quickly after prolonged exposure to the ligand) than wild type at -100mV, as well as significantly higher sensitivity to ACh; while the α4 subunit S252L mutation displayed higher sensitivity to ACh than wildtype, and yet only homozygously expressed S252L showed significantly lower maximal ACh-evoked currents (Bertrand et al., 2002). They also addressed the finding that α4 mutant expressing receptors are more desensitized than control receptors, and showed that heterozygous α4S248F and α4S252L mutant expression failed to display significant changes in desensitization (Bertrand et al., 2002). Previous research showed that α4S248F and α4L776ins3 mutants display decreased calcium permeability, so they addressed ion selectivity in α4S252L. Cells expressing α4S252L in a heterozygous manner showed similar dependence of reversal potential versus the extracellular calcium concentration, as well as similar amplitudes and time course ACh-evoked currents when compared to wildtype (Bertrand et al., 2002).
Acetylcholine in the intermembrane space is metabolized by ACh-esterase, yielding choline as a byproduct. Choline may act as a nAChR agonist, so another possible mechanism for abnormal nAChR physiology could be whether mutant receptors show altered choline activation. The α4 subunit S248F and S252L mutations, and β subunit V287M mutation, but not the α4L776ins3 mutation, showed significant alteration in receptor profile compared to wild type, where choline acted as a partial agonist (Bertrand et al., 2002). While each CHRNA4 and CHRNB2 mutation has distinct physiological properties that need to be further examined, all mutations increase the receptor ACh sensitivity (Bertrand et al., 2002). Increased receptor sensitivity supports the hypothesis that a gain-of-function mutation may play a role in offsetting the balance in the communication loop between cortical and thalamic systems.
Cortical pyramidal cells and thalamic reticular nucleus neurons relay signals in a balanced loop. Cortical pyramidal cells are dual action cells and cholinergic fibers activate the dendrites of pyramidal cells, which may counteract signals directed from the cortical layer to the thalamus. This would suggest that a gain-of-function nAChR receptor mutation decreases the input to the thalamus from external cortical layers. By reducing feedback from the external layers, thalamo-to cortical signals would be reinforced, sending out more excitatory signals to the cortex. Thus defective nAChR may disrupt the balance between circuit excitation and inhibition, promoting seizure formation (reviewed in Steinlein & Bertrand, 2010). This perturbed thalamocortical loop would help to directly explain the distinct sleep-associated phenotype of ADNFLE seizures. Despite advances in research, the mechanism behind increased ACh sensitivity is still poorly understood (Kullmann, 2010).
Voltage –gated sodium channels
Voltage-gated sodium channels act as a gate for the diffusion of sodium cations across the plasmalemma. At resting potential, extracellular sodium concentration is 10 times higher than the concentration inside a neuron, and the outer gate of the channel is closed. The closed position of the channel is termed its “deactivated” state. When the channel is subjected to a positive current, the charge induces conformational changes of the channel pore, opening the outer gate and “activating” the channel. As the gate opens, it provides a passage for an influx of sodium ions. This brief influx of positive charge flips the membrane polarity, and as the inner membrane passes the threshold level, the cell is depolarized and propagates an action potential. Within a fraction of a millisecond, the inner gate closes to stop sodium from entering the neuron, and the channel is inactivated. The channel’s direct role in generating and propagating action potentials across an entire neuron makes it a critical protein in maintaining functional neuronal electricity. Mutations in both of the sodium channel subunits, the pore-forming α subunit and auxiliary β subunit (encoded by nine and four genes respectively,) are linked to epilepsy (reviewed in Reid, et al., 2009). More than 300 dominant mutations have been identified on the SCN1A gene (Kullmann, 2010).
In 1998, Wallace et al. identified the first mutation in a sodium channel linked to generalized epilepsy with febrile seizures plus (GEFS+). GEFS+ is an inheritied epilepsy syndrome of generalized febrile and afebrile seizures that may extend beyond childhood ages, when febrile seizures typically stop occurring (Scheffer & Berkovic, 1997). The identified C121W mutation was located in the β1 subunit gene SCN1B, in a region on the extracellular domain that is significant for protein function (Wallace et al., 1998). The mutation disrupts a highly conserved codon responsible for a disulfide cysteine bridge in the extracellular domain (Wallace et al., 1998). Therefore the hypothesis regarding β subunit mutations suggested that disruptions of the extracellular domain result in muted sodium channel expression, slower inactivation and slower recovery from inactivation.
In order to identify some of the biophysical properties of the mutant gene, the mutant and wild type SCN1B were co-expressed with the rat brain α subunit in Xenopus laevis oocytes, and electronic functioning of the channels were measured with two-electrode voltage clamp recordings (Wallace et al., 1998). The study showed that co-expression of WT α subunit with mutant SCN1B causes sodium channels to inactivate at a significantly slower rate, consistent with the loss-of-function phenotype in GEFS+ families.
Studies continued and in 2007, Xu et al. identified two mutations in the β1 subunit in patients with GEFS+. The mutations were on the extracellular immunoglobulin-like domain, further supporting the importance of this extracellular domain. In order to characterize any mutant alterations on channel physiology, human brain α subunit NaV1.2 was expressed alone, as well as co-expressed with wildtype and mutant β1 subunits in a human embryonic kidney (HEK-293T) model, and patch clamp methods were used (Xu et al., 2007a). Cells expressing the mutant β1 subunit showed inhibited voltage dependence of activation, reduced dependence, and fast and slow inactivation (Xu et al., 2007a). One of the mutant forms, R85C, showed no modulating effects compared to the α subunit NaV1.2 alone, while the other mutant (R85H) also failed to modulate fast kinetics, it did inhibit the voltage dependence of steady state slow inactivation similar to wild type (Xu et al., 2007a). Immunohistochemistry was also used to identify the location of the β1 subunits, and showed that the wildtype subunit localized on the cell membrane, while mutant forms did not (Xu et al., 2007a). These studies supported the underlying mechanism that sodium channel subunit mutations lead to phenotypic, that is, mutations fail to modulate the pore-forming alpha subunit, leading to electrophysiological abnormalities. In humans with epilepsy, the underlying mechanism of GEFS+ sodium channel mutations are due to loss-of-function mutations, which leads to hyperexcitability by increasing the transmembrane sodium influx. Because the sodium channel is responsible for instigating and propagating the action potential, these data indicate that epileptic hyperexcitabilty is contingent upon the tight regulation of ion diffusion. These data also helped contribute to the current understanding that epilepsy is a channelopathy.
Voltage-gated potassium channels
Similar to voltage-gated sodium channels, voltage-gated potassium channels play a critical role in the conductance of action potentials. Potassium channels are the most widely expressed ion channel and over 70 subunits have been identified to date. At resting potential, the intracellular concentration of potassium cations is maintained to be twenty times higher compared to the extracellular environment. Potassium channels are less sensitive than sodium channels, so they open subsequently to sodium channels. The potassium channel has one gate, and when the protein changes conformation, the gate opens, allowing potassium to surge out of the neuron. This efflux of positive charge repolarizes the neuron during an action potential, and when the potassium channel closes, the resting potential is reestablished. Fully functional potassium channels repolarize the membrane in a timed fashion, thereby regulating electrical conductance.
Voltage-gated potassium channels called M-channels have a different physiological role. M-channels are partially active during the resting membrane potential and are further activated upon depolarization. They open and close very slowly compared to other ion channels, and serve to oppose sustained membrane depolarization and repetitive firing of action potentials (Cooper & Jan, 2003). Broadly, mutations in the potassium channels lead to disruption of neuronal firing patterns, altered resting membrane potential and increased calcium influx and neurotransmitter release (reviewed in Reid et al., 2009). These effects suggest a mutation in potassium channels could follow many pathways to hyperexcitability and the epileptic phenotype.
In 1998. Biervert et al. identified the third idiopathic inherited gene defect, which was linked to benign familial neonatal convulsions (BFNC), a rare autosomal dominant infancy epilepsy. BFNC is characterized by frequent, unprovoked partial or generalized clonic convulsions, which occur during wakefulness or sleep. Seizures begin within the first days after birth and usually cease within a number of weeks or months, but extend later into life for 10-15% of affected children. The team identified a five-base pair insertion that would prematurely end the KCNQ2 potassium channel Kv7.2 subunit, leaving out over 300 amino acids (Biervert et al., 1998). To identify functional abnormalities of the mutant, they injected wild type and truncated KCNQ2 complementary RNA in Xenopus laevis oocytes, and used two-electrode voltage clamps for analysis (Biervert et al., 1998). The wild type form displayed a current that began to activate above -60 mV and was fully activated at 0 mV, while the truncated form displayed currents like those of the negative control, and a heterozygous model (with equivalent mutant and wild type coinjection) showed reduced currents compared to cells injected with equivalent amounts of only wild type cRNA (Biervert et al., 1998). These studies were the first to link potassium channels to human epilepsy, and although the results had no clear dominant negative effect, they proved to lead a list of studies that would show haplosufficiency is sufficient for the observed phenotype.
The study also paved the hypothesis that defects in membrane repolarization can be a causative mechanism of epilepsy. Again, the evidence was mounting that epilepsies are channelopathies, as each of the first three inherited idiopathic epilepsy mutations were located within the coding sequence of ion channel subunits. Around the time of the study by Biervert et al., Charlier et al. identified a mutation in the potassium subunit KCNQ3 (1998), and shortly thereafter Wang et al. identified both KCNQ2 and KCNQ3 as M-channels (1998). Activation of M-channels serves to stabilize membrane potential and limit action potential firing. Specifically, KV7/M-type potassium channels activate near the threshold of action potential without significantly inactivating, and antagonize repetitive firing of neurons during persistent depolarizing inputs (Delmas & Brown, 2005). Based on this property, it was hypothesized that epilepsy mutations disrupting M-channel functioning would increase neuronal excitability.
Additional studies showed that mutations in M-current potassium channel subunits reduce the size of potassium currents by 20-95%, indicating that a modest reduction (20%) in KCNQ channel activity could lead to the epilepsy phenotype (reviewed in Cooper , 2003). Recent work has further characterized the intricacies of M-channel electrophysiology. Wuttke et al. identified a novel BNFC-causing mutation (E119G) in the conserved S1-S2 region of KCNQ2-a region not previously implicated in KCNQ mutant studies (2008). Using a Xenopus laevis oocyte model, and a standard two-microelectrode voltage clamping method, they coexpressed the E119G mutant alone, and with wild type KCNQ2, KCNQ3 and KCNQ2+KCNQ3, as well as controls of KCNQ2 and KCNQ2+KCNQ3 (Wuttke, 2008). Compared to wild type controls, expression of the E119G mutant caused a shift toward depolarized voltages, reduced the relative current amplitudes at the subthreshold range of -50mV, and significantly slowed activation kinetics (Wuttke et al., 2008). By conducting computer-modeling analysis, they showed that the S1-S2 domain might interact with a positive charge on the S4 region, further implicating the structure to function relationship (Wuttke et al., 2008). Their study implicated the importance of very stringent M-channel regulation. It also showed that at subthreshold levels, very minimal changes in channel gating could be enough to induce neonatal seizures. Therefore M-channel malfunction plays a significant role in leading to seizures.
Ligand-gated GABAA receptors
GABA is the most prevalent inhibitory neurotransmitter and serves to hyperpolarize neurons, decreasing the ability to generate an action potential. Based on pharmacological studies in animal models, it was known for some time that GABA plays a role in epilepsy. In 2001, the first mutations identified in the GABAA receptor were located within the γ2-subunit (GABRG2). The GABAA and GABAC receptors are ionotripic while GABAB receptor is metabotropic; so far, mutations in GABAA receptor subunits alone (GABRG2 and GABRA1) have been identified in epilepsy (reviewed in Reid et al., 2009). Receptor proteins were of high interest to study because if a mutation inhibits receptor function, a postsynaptic neuron may not receive inhibitory signals, as it should. The R43Q mutation was identified in individuals with childhood absence epilepsy and febrile seizures, and the K289M mutation was identified in individuals with GEFS+ (Wallace et al., 2001; Baulac et al., 2001). The K289M mutation is located in the extracellular loop between M2 and M3, and mutant expression in Xenopus laevis oocytes displayed inhibited GABA-activated currents, supporting the loss-of-function hypothesis (Baulac et al., 2001). On the other hand, the R43Q mutant expression in Xenopus laevis oocytes showed no current changes elicited by GABA, yet entirely lost the ability to produce a current elicited by the drug diazepam (Wallace et al., 2001). Because the R43Q mutant form successfully assembled with other subunits and displayed typical zinc blockade sensitivity, the researchers concluded that the R43Q mutation functionally lacks diazepam potentiation, which would suggest a role for native endozepine molecules in preventing both febrile seizures and absence seizures (Wallace et al., 2001).
In an effort to understand the neuronal mechanisms altered by GABAA mutations, Eugène et al. honed in on the mediations of GABRG2 mutations in both phasic and tonic inhibition (2007). Phasic inhibition is induced by vesicle release, leading to fast GABA inhibition, while persistent, tonic inhibition occurs with receptors remote from synapses, and is elicited from low concentrations of ambient GABA. The team transfected murine hippocampal cultures with GFP-tagged mutant GABRG2, and showed that two mutant γ subunits (K289M, R43Q) have unique properties. The K289M mutation, which is linked to GEFS+, accelerated deactivation of synaptic GABA currents in a dominant manner, but did not alter tonic GABA currents (Eugène et al., 2007). The mutation also had no effect on trafficking or aggregation of the GABAA receptor (Eugène et al., 2007). The R43Q mutation, which is linked to CAE and febrile seizures, caused reduced tonic currents, but did not affect the synaptic kinetics (Eugène et al., 2007). This mutant form also showed differential surface expression and synaptic aggregation compared to the wild type γ subunit, which may be due to homo-oligomer aggregation, but the effective density of functional receptors was not decreased because the receptor maintained its miniature inhibitory post synaptic current (mIPSC) (Eugène et al., 2007).
The study indicated that two GABRG2 mutations alter GABA-ergic signaling in very distinct ways. Namely, they suggest that the K289M mutation reduces phasic inhibition and R43Q reduces tonic inhibition (Eugène et al., 2007). GABAA receptor defects are not unimodal, and different abnormalities may lead to the common effect of reduced GABA inhibition. A new target will be to characterize the role of phasic and tonic inhibition with other mutants as well as identify more defined molecular effects in animal models.
Glial Cells
Within the last ten to fifteen years, studies have begun to look at the role of astrocytes in epilepsy in a number of pathways. These include modulation of neuronal excitability via astrocytic release of glutamate, pro- and anticonvulsant roles of cytokines released by reactive astrocytes, glutamine synthetase dowregulation, and water and potassium balance in reactive astrocytes (see Wetherington et al., 2008).
Astrocytes are the most abundant cell type in the human brain and have many functions, including nutritive functions, ion buffering, releasing chemicals and maintaining the blood brain barrier. And very importantly, astrocytes have a primary role in glutamate uptake to clear the synapse after neurotransmitter release. Any disturbance in this function would directly yield excessive glutamate accumulation in synaptic clefts, and lead to hyperexcitability. Additionally, animal models of epilepsy as well as mesial temporal lobe epilepsy begin reactive gliosis, indicating these cells are activated in epilepsy (reviewed in Wetherington, Serrano, & Dingledine, 2008).
One interesting study showed that glial cells may play a role that promotes or inhibit the epilepsy phenotype. Interleuikin (IL)-1β is a proinflammatory cytokine implicated in neuron excitability. It has corresponding type I and type II receptors (IL-1R) and receptor antagonist IL-1Ra, all of which are upregulated after induced convulsions in rodent models. It was shown that an increased production of IL-1β in microglia-like cells of the rat hippocampus as well as direct addition of exogenous IL-1β prolongs EEG seizures by increasing glutamatergic transmission (Vezzani et al., 1999). Therefore, Vezzani et al. hypothesized that IL-1R antagonist may play a role for counteracting seizures in rodents. They showed that by introducing recombinant IL-1Ra in the brain or overexpressing endogenous IL-1Ra in astrocytes of various mice strains, seizure convulsions were highly delayed and reduced, based on behavioral and EEG testing (Vezzani et al., 2000). The type 1 receptor was identified to mediate the observed antagonist effects, as IL-1Ra had no effect in IL-R1-type one knockout mice (Vezzani et al., 2000). Thus IL-IRa has a potent anticonvulsant property. The IL-1β cytokine enhances glutamate transmission, so it would follow that IL-IRa inhibits the glutamate transmission. This implicated mechanism directly plays a role in glutamate-induced hyperexcitability that is characteristic of epilepsy; hence, cytokine activity in astrocyte may play a significant role in modulating seizure phenotypes. The balance between cytokines and their agonists can be altered to generate convulsants or anticonvulsant properties. More specifically, increasing the IL-1Ra /IL-1β ratio may provide a valuable method for inhibiting seizures.
Treatment
The goal in epilepsy treatment is to relieve patients from seizures so that they live without the fear of an approaching seizure. In the 1920’s the ketogenic diet was developed as a treatment for epilepsy. This diet consists of mainly fatty foods, limited protein, and minimal carbohydrates. This encourages ketosis, similar to the effects of fasting on the body. Although the therapeutic mechanism behind this diet is unclear, it proves to decrease seizures in a non-invasive and drug-free way. Surgery to remove epileptic neurons has been a useful method to relieve seizures, and is still a very viable treatment option. In vagus nerve stimulation (VNS) therapy, the vagus nerve is connected to a subcutaneously placed generator by electrodes. The generator supplies regular nerve stimulation and seizures can be stopped by magnetizing the generator, but it is not understood how this therapy works. This treatment is costly and requires surgery.
Drug therapy
Refer to Figure 2 for an overview of drug therapy in epileptic cells. By 1978 there were a number of effective, relatively affordable antiepilepsy drugs (reviewed in Leeman & Cole, 2008). These include carbamazepine, phenobarbital, phenytoin, primidone, ethosuximide. Since 1993, nine drugs have been approved by the U.S. FDA, which many have lesser side-effects, including: felbamate, gabapentin, topiramate, lamotrigine, tiagabine, levetiracetam, zonisamide, oxcarbazepine, and pregabalin. Felbamate is a NMDA receptor antagonist and was approved in 1993 (Leeman & Cole, 2008). Gabapentin resembles GABA, but it does not work by a GABA-ergic mechanism. The mechanism of the drug is unclear, but may work by binding voltage-gated calcium channels. Topiramate works by a number of mechanisms, including blocking voltage-gated sodium channels, antagonizing AMPA/kainite glutamate receptors, modulating GABAA-mediated chloride activity and inhibiting carbonic anhydrase. The mechanism behind lamotrigine therapy is unclear, but may be due to inhibiting sodium channels. Tiagabine works by inhibiting GABA reuptake into neurons and glia. The mechanism behind levetiracetam therapy is unclear, but it has been shown to bind SV2A, a synaptic vesicle protein. Zonisamide works to stabilize membranes by blocking sodium and T-type calcium channels. Oxcarbazepine works by blocking voltage-sensitive sodium channels. The newest anticonvulsant drug is pregabalin, and is structurally similar to GABA, but does not work by a GABA-ergic mechanism (similar to gabapentin). By binding calcium channels, Pregabalin reduces calcium influx and inhibits the release of neurotransmitters including glutamate.
Future Therapy
New drugs are currently being developed to modulate sodium channels, potassium channels and chloride channels, and a number are now going through clinical trials. Cutting edge research also includes photoregulation of GABAA-receptor elicited responses like cell membrane current and spike-firing rate, using light in the visible spectrum (Yue et al., 2012).
Conclusion
Epilepsy is a very diverse and heterogeneous brain disorder. It is not limited to any cause, age, or seizure type. Beginning with the identification of the first inherited mutation in the ligand-gated nAChR, epilepsy has been classified as a channelopathy. Yet the disease mechanism is not limited to ion channels alone. Research has characterized many attributes of the mechanisms that contribute to specific seizure syndromes, but a lot has yet to be discovered. Current research is very promising, and future research may provide an answer as to what unifying mechanisms cause seizure instigation. There are many therapy options available for people with epilepsy and as research advances, treatment will become more effective, less expensive, and elicit less side-effects.
Acknowledgements: I would like to thank Dr. DebBurman for continued academic support and enrichment. I would also like to thank the Sajan Koirala and the entire Bio362 class for their encouragement and support.
References
Baulac, S., Huberfeld, G., Gourfinkel-An, I., Mitropoulou, G., Beranger A., Prud’homme, J. F., Baulac, M., Brice, A., Bruzzone, R., & LeGuern E. (2001). First genetic evidence of GABAA receptor dysfunction in epilepsy a mutation in the γ2-subunit gene. Nat. Genet., 28, 46–48.
Biervert, C., Schroeder, B. C., Kubisch, C., Berkovic, S. F., Propping, P., Jentsch, T. J., Steinlein, O. K., 1998. A potassium channel mutation in neonatal human epilepsy. Science 279, 403–406.
Bizière, K., & Chambon, J. P. (1987). Animal models of epilepsy and experimental seizures. Revue neurologique, 143(5), 329.
Buchhalter, J. R. (1993). Animal models of inherited epilepsy. Epilepsia, 34, S31-S41.
Charlier, C., Singh, N. A., Ryan, S. G., Lewis, T. B., Reus, B. E., Leach, R. J., & Leppert, M. (1998). A pore mutation in a novel KQT-like potassium channel gene in an idiopathic epilepsy family. Nature genetics, 18(1), 53-55.
Cooper, E. C., & Jan, L. Y. (2003). M-Channels-: Neurological diseases, neuromodulation, and drug development. Arch Neurol, 60(4):496-500. doi:10.1001/archneur.60.4.496.
Delmas, P., & Brown, D. A. (2005). Pathways modulating neural KCNQ/M (Kv7) potassium channels. Nature Reviews Neuroscience, 6(11), 850-862.
Devillers-Thiery, A., Galzi, J. L., Eisele, J. L., Bertrand, S., Bertrand, D., & Changeux, J. P. (1993). Functional architecture of the nicotinic acetylcholine receptor: a prototype of ligand-gated ion channels. Journal of Membrane Biology, 136(2), 97-112.
Epilepsy Foundation. (n.d.). Partial vs. Generalized Epilepsies. Retrieved November 28, 2012 from http://www.epilepsyfoundation.org/aboutepilepsy/causes/partialgeneralized.cfm.
Epilepsy. (2004). Epilepsy Therapy Project: History of Epilepsy. Retrieved November 28, 2012 from http://www.epilepsy.com/epilepsy/history
Eugène, E., Depienne, C., Baulac, S., Baulac, M., Fritschy, J. M., Le Guern, E., Miles, R., & Poncer, J. C. (2007). GABAA receptor γ2 subunit mutations linked to human epileptic syndromes differentially affect phasic and tonic inhibition. The Journal of Neuroscience, 27(51), 14108-14116.
Helbig, I., Scheffer, I. E., Mulley, J. C., & Berkovic, S. F., (2008). Navigating the channels and beyond: Unravelling the genetics of the epilepsies. Lancet Neurol. 7, 231–245.
Kullmann, D. M. (2010). Neurological channelopathies. Annual review of neuroscience, 33, 151-172.
Leeman, B. A., & Cole, A. J. (2008). Advancements in the treatment of epilepsy. Annu. Rev. Med., 59, 503-523.
Löscher, W. (1984). Genetic animal models of epilepsy as a unique resource for the evaluation of anticonvulsant drugs. A review. Methods Find Exp Clin Pharmacol, 6(9), 531-47.
PubMed Health. (2012). Epilepsy. Retrieved November 28, 2012 from http://www.ncbi.nlm.nih.gov/pubmedhealth/PMH0001714/
Ramos-Mandujano, G., Vazquez-Juarez, E., Hernandez-Benitez, R., & Pasantes-Morales, H. (2007). Thrombin potently enhances swelling-sensitive glutamate efflux from cultured astrocytes. Glia 55, 917–925.
Reid, C. A., Berkovic, S. F., & Petrou, S. (2009). Mechanisms of human inherited epilepsies. Progress in neurobiology, 87(1), 41-57.
Scheffer, I. E., Bhatia, K. P., Lopes-Cendes, I., Fish, D. R., Marsden, C. D., Andermann, E., Andermann, F., Desbiens, R., Keene, D., Cendes, F., Manson, J., Canstantinou, J. E. C., McIntosh, A., & Berkovic, S. F. (1995). Autosomal dominant nocturnal frontal lobe epilepsy a distinctive clinical disorder. Brain, 118(1), 61-73.
Scheffer, I. E. & Berkovic, S. F. (1997). Generalized epilepsy with febrile seizures plus. A genetic disorder with heterogeneous clinical phenotypes. Brain 120, 479−490.
Steinlein, O. K. (2004). Genetic mechanisms that underlie epilepsy. Nature Reviews Neuroscience, 5(5), 400-408.
Steinlein, O. K., & Bertrand, D. (2010). Nicotinic receptor channelopathies and epilepsy. Pflügers Archiv European Journal of Physiology, 460(2), 495-503.
Steinlein, O. K., Mulley, J. C., Propping, P., Wallace, R. H., Phillips, H. A., Sutherland, G. R., Scheffer, I. E., Berkovic, S.F. (1995). A missense mutation in the neuronal nicotinic acetylcholine receptor alpha 4 subunit is associated with autosomal dominant nocturnal frontal lobe epilepsy. Nat. Genet. 11, 201–203.
Steriade, M., McCormick, D. A., & Sejnowski, T. J. (1993). Thalamocortical oscillations in the sleeping and aroused brain. Science, 262, 679-679.
Vezzani, A., Conti, M., De Luigi, A., Ravizza, T., Moneta, D., Marchesi, F., & De Simoni, M. G. (1999). Interleukin-1β immunoreactivity and microglia are enhanced in the rat hippocampus by focal kainate application: Functional evidence for enhancement of electrographic seizures. The Journal of neuroscience, 19(12), 5054-5065.
Vezzani, A., Moneta, D., Conti, M., Richichi, C., Ravizza, T., A., De Simoni, M. G., Sperk, G., Andell-Jonsson, S., Lundkvist, J., et al. (2000). Powerful anticonvulsant action of IL-1 receptor antagonist on intracerebral injection and astrocytic overexpression in mice. Proc. Natl. Acad. Sci. USA 97, 11534–11539.
Wallace, R. H., Marini, C., Petrou, S., Harkin, L. A., Bowser, D. N., Panchal, R. G., Williams, D. A., Sutherland, G. R., Mulley, J. C., Scheffer, I. E., & Berkovic, S. F. (2001). Mutant GABAA receptor γ2-subunit in childhood absence epilepsy and febrile seizures. Nature Genetics, 28(1), 49-52.
Wallace, R. H., Wang, D. W., Singh, R., Scheffer, I. E., George, A. L., Phillips, H. A., Saar,K., Reis, A., Johnson, E. W., Sutherland, G. R. & Mulley, J. C. (1998). Febrile seizures and generalized epilepsy associated with a mutation in the Na+-channel ß1 subunit gene SCN1B. Nature genetics, 19(4), 366-370.
Wang, H. S., Pan, Z., Shi, W., Brown, B. S., Wymore, R. S., Cohen, I. S., Dixon, J. E., & McKinnon, D. (1998). KCNQ2 and KCNQ3 potassium channel subunits: molecular correlates of the M-channel. Science, 282(5395), 1890-1893.
Wetherington, J., Serrano, G., & Dingledine, R. (2008). Astrocytes in the epileptic brain. Neuron, 58(2), 168-178.
Wuttke, T. V., Penzien, J., Fauler, M., Seebohm, G., Lehmann‐Horn, F., Lerche, H., & Jurkat‐Rott, K. (2008). Neutralization of a negative charge in the S1–S2 region of the KV7. 2 (KCNQ2) channel affects voltage‐dependent activation in neonatal epilepsy. The Journal of Physiology, 586(2), 545-555.
Xu, R., Thomas, E.A., Gazina, E.V., Richards, K.L., Quick, M., Wallace, R. H., Harkin, L. A., Heron, S. E., Berkovic, S. F., Scheffer, I. E., Mulley, J. C., Petrou, S., 2007a. Generalized epilepsy with febrile seizures plus-associated sodium channel beta1 subunit mutations severely reduce beta subunit-mediated modulation of sodium channel function. Neuroscience 148, 164–174.
Yue, L., Pawlowski, M., Dellal, S. S., Xie, A., Feng, F., Otis, T. S., Bruzik, K. S., Qian, H., & Pepperberg, D. R. (2012). Robust photoregulation of GABAA receptors by allosteric modulation with a propofol analogue. Nature Communications, 3, 1095.