Catalase overexpression rescues Friedreich’s Ataxia mouse models from oxidative stress and mitochondrial iron-loading
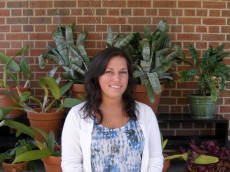
Daniella Brutman and Menzi Mhlanga
Department of Biology
Lake Forest College
Lake Forest, Illinois 60045
Download PDF
Abstract
Friedreich’s ataxia (FRDA) is an inherited neurodegenerative disorder characterized by gait disturbance and speech problems. Disease pathology is characterized by progressive damage and loss of nerve tissue particular to the peripheral nerve system. FRDA is caused by the relative deficiency of a mitochondrial protein frataxin resulting from an expanded intronic GAA triplet repeat. While the precise role of frataxin has been the subject of controversy for the past decade, it is well accepted that FRDA pathology is related to mitochondrial dysfunction. Yeast and Drosophila models of FRDA exhibit signs of oxidative damage which has led us to the hypothesis that catalase over expression will rescue frataxin deficient peripheral neuronal cells and that H2O2 plays a critical role in FRDA pathogenesis. Furthermore, by eliminating the cell content of H2O2 we can alleviate some of the symptoms of FRDA. To test our hypothesis, we aim to i) generate a conditional knockout mice expressing frataxin deficiency in the nervous system as our model for studying hydrogen peroxide toxicity, ii) manipulate cell content of H2O2 by modulating the amount of catalase and iii) investigate the regulation of mitochondrial iron loading proteins in the same model system.
Background
Many neurodegenerative diseases are similar in the mode of action, pathology, and etiology, and these parallels include induced cellular death in various levels of neuronal circuitry (1). Oxidative stress and mitochondrial dysfunction are two central features shared amongst most neurodegenerative diseases (2). Friedreich’s ataxia is one of the many neurodegenerative diseases believed to be connected to both mitochondrial dysfunction and oxidative stress.
Friedreich’s ataxia (FRDA) has a prevalence rate of 1 in 50,000 people affected with the autosomal recessive disease, making it one of the most common forms of ataxia. FRDA begins with the degeneration of larger sensory neurons, typically in the dorsal root ganglia. This usually happens around puberty, and FRDA onset always occurs before the age of 25 (4). FRDA’s pathology is characterized by the progressive loss of sense of position, tendon reflexes, limb coordination, dysarthia nystagmus, hypertrophic cardiomyopathy, and even diabetes in about 20% of cases (5-7). In fact, over half of FRDA patients die at an early age from cardiac-related complications (6). Tissues with higher metabolic rates, such as the liver and skeletal muscles, are affected more in FRDA patients, but peripheral nervous system cells and cardiac cells are those affected most (8).
The mutation for FRDA was discovered on chromosome 9q13q21.1, specifically on gene X25, otherwise known as Frataxin (8). This intronic GAA trinucleotide expansion, which codes for glutamic acid, causes the number of GAA repeats, which is usually around 7-22 in healthy individuals, to increase to 700-800 repeats, with the highest X25 expression being in the heart tissue (8, 33). This GAA expansion induces a triple helical structure during the transcription of the protein, which affects the transcription rate. Therefore, the longer the repeat is, the greater the frataxin deficiency (9-12). While frataxin’s specific function in the cell is not completely understood, it is believed to play a role in heme biogenesis, the ISC synthesis pathway, mitochondrial iron storage, intracellular iron trafficking, the prevention of the formation of reactive oxygen species (ROS), and the regulation of aconitase (5,6, 13-15).
Oxidative stress is believed to be a major contributing factor to the pathology of FRDA, and the mitochondrial iron loading found in FRDA patients is being studied as a contributing factor (16, 17). Along with contributing to mitochondrial iron loading, frataxin also plays a role in the prevention of the formation of deleterious reactive oxygen species (ROS), which may be further increased by mitochondrial iron loading, and thus, leads to increased mitochondrial dysfunction and cell death (4, 17-20). This problem is furthered by reduced aconitase activity associated with FRDA pathology that causes an increase in the transcription of iron-import mechanisms and increases oxidative stress due to aconitase’s role in oxidation during the Krebs-cycle (14, 21- 23). Iron, is very reactive in oxygen-based redox chemistry, which explains the correlation between iron metabolism deregulation and increased oxidative stress; in yeast, iron plays a role in ferroxidase reactions where H2O2 is generated through the consumption of oxygen (14, 24, 25).
A reduction in aconitase activity and increase in H2O2 concentrations in Drosophila FRDA models is believed to contribute to FRDA pathogenesis (26). Furthermore, it has been discovered that catalase deficient Drosophila exhibited more FRDA symptoms and shorter life spans, and Drosophila with over expressed catalase exhibited reduced symptoms and longer lifespan (26). Anderson et al. (2008) found that catalase was able to rescue FRDA cells from cell death due to H2O2 toxicity by increasing the amount of H2O2 converted to H2O rather than OH-. The decrease in OH- reduces oxidative stress (26). The effect of catalase has yet to be tested in more advanced mouse models, which leads to the gap in knowledge in the field of FRDA: can the effects of frataxin deficiency on oxidative stress and mitochondrial iron loading be reversed in frataxin deficient mice through the over expression of catalase? We hypothesize that catalase over expression will rescue frataxin deficient peripheral neuronal cells and that H2O2 plays a critical role in FRDA pathogenesis. We will assess this hypothesis by i) developing a mouse model of Friedreich’s ataxia and characterizing FRDA associated components with respect to WT mice, ii) investigating the role of hydrogen peroxide in catalase deficient and catalase augmented FRDA conditional knockout and WT mice, and iii) characterizing the role of aconitase and other iron metabolism regulating genes in FRDA conditional knockout and WT mice.
Significance
Broader Relevance
FRDA is the most prevalent of all ataxias (36). Friedreich’s ataxia is accompanied by various forms of heart disease, such as hypertrophic cardiomyopathy and diabetes (36, 37). Although these conditions are manageable, there is currently no available treatment for FRDA. Patients affected by the disease ultimately succumb to death as a result of heart failure and/or diabetes. It is therefore necessary to find a therapeutic protocol targeted specifically at FRDA patients to alleviate, if not cure, the symptoms of FRDA. The findings of this proposal will reinforce the need to target hydrogen peroxide as a means to alleviate disease burden in FRDA patients. Moreover, the study of FRDA in our laboratory will provide undergraduate students with the opportunity to conduct undergraduate research: gaining practical knowledge of research techniques and the necessary skills.
Intellectual Merit
The aim of our proposal is to study the role of hydrogen peroxide in a mouse model of FRDA. This will enable us to develop a therapeutic model that will be subsequently used in drug treatments for the disease. Studying the role of hydrogen peroxide with relation to FRDA will enable us to also get a more precise model for the mechanisms underlying disease pathology and frataxin loss. Whereas other models propose an iron accumulation based model of FRDA (38-40), we predict that the findings of this proposal will serve as a re-enforcer to the hypothesis that FRDA pathology is a result of oxidative damage more than any other feature of frataxin deficiency. Mitochondria are the primary location of FRDA pathology. It is likely that studying mechanisms related FRDA will provide new insights into the overall machinery of the mitochondria, advancing our knowledge of sub-cellular reactions of the mitochondria.
Specific Aims
The aim of our proposal is to replicate the findings of Anderson et al. (2007) in a mammalian model of the disease using conditional knockout mice (17). Consistent with Anderson et al. (2007), we hypothesize that hydrogen peroxide plays an important role in FRDA symptomatology. We predict to find a positive relationship between hydrogen peroxide concentration and FRDA symptomatology as moderated by the enzyme catalase. Thus scavenging for hydrogen peroxide will increase lifespan and alleviate some symptoms in frataxin conditional knockout mice.
Our aims for our hypothesis are as follows.
1. Develop a mouse model of Friedreich’s ataxia and characterize FRDA associated components with respect to WT mice: Mice lacking frataxin in neuronal cells will be developed using cre-lox recombination (17). Characteristics such as gait, posture, lifespan, as well as indicators of oxidative stress will be determined.
2. Investigate the role of hydrogen peroxide in catalase deficient and catalase augmented FRDA conditional knockout and WT mice: To study the effects of hydrogen peroxide availability in cells, conditional knockout mice lacking frataxin in neuronal cells will be crossed with i) catalase over expressing mice to produce a line of mice simultaneously over expressing catalase in all somatic cells and lacking the protein frataxin in neuronal cells and ii) catalase knockout mice to produce a line of mice simultaneously lacking catalase in all somatic cells and frataxin in neuronal cells.
3. Characterize the role of aconitase and other iron metabolism regulating genes in FRDA conditional knockout and WT mice: Catalase will be over expressed and silenced in the peripheral neuronal cells of frataxin knockout mice, and we will investigate the expression of aconitase and 10 iron regulating genes found to have altered expression in previous studies of frataxin knockout cardiac cells (27).
Research Methods & Design
1. Develop a mouse model of Friedreich’s ataxia and characterize FRDA associated components with respect to WT mice
Rationale: Frataxin knockout mice do not survive beyond the embryonic stage (17). Therefore, we intend to replicate the methodology used by Puccio et al (2001) to develop a conditional knockout mouse model of FRDA. While this method produces a striated muscle deficient line (MCK), we intend to use a neuron specific line because of its functional relevance to human FRDA, as peripheral neuron degradation is the main pathological feature of human FRDA. We hypothesize that hydrogen peroxide is critical to the underlying pathogenesis of FRDA. Therefore, we suspect that oxidative damage will be significantly higher in conditional knockout mice compared to WT mice.
Design and Method
Generating a conditional knockout mouse model:
To generate our mouse model of FRDA, we will replicate the methodology used by Puccio et al. (2001) to generate a neuron frataxin deficient line of mice. As noted above, global deficiency of frataxin in mice results in embryonic death (17). Using the cre-lox recombination technique, we intend to generate a conditional knockout mouse model lacking frataxin expression in the nervous system.
To obtain a viable model, mice homozygous for the Frda allele will be crossed with mice heterozygous for the deletion of exon 4 in the Frda allele carrying a tissue specific Cre-transgene under the control of neuron specific enolase (NSE). A targeting vector will be electroplated into embryonic cells, after which DNA resistant clones will be analyzed by PCR using the frataxin primer to generate a correct fragment for targeted embryonic stem cells. Targeted stem cells will be injected into host blastocysts from pseudo-pregnant female mice (17). To confirm frataxin deficiency in conditional knockout (KO) mice, nerve tissue extracted from a peripheral neuron will be used to determine frataxin expression using RT-PCR as described in ref. 17.
Next, characterization of mice will be carried out to note such characteristics as movement, gait, posture, weight, and lifespan in all chimeras. Moreover, a Pole test and a Stride Length Test will be used to characterize movement stability in chimera mice vs. WT mice.
Prediction: We predict that NSE chimeras will exhibit severe signs of movement disorders, gait, abnormal posture and a very short lifespan. Moreover, we predict conditional KO mice to perform poorly in the Pole Test and the Stride Length Test compared to WT mice. If conditional KO mice do not exhibit any of the above listed symptoms, this would suggest that frataxin KO in mice neuronal cells is not an adequate model of FRDA.
Determination of cellular hydrogen peroxide activity:
To determine whether mitochondria from conditional knockout mice exhibit high levels of hydrogen peroxide production, we will carry out an assay for intra-mitochondrial hydrogen peroxide concentration using mitochondria isolated from nerve tissue of both WT and conditional knockout mice (23). In order to obtain nerve tissue, both WT and conditional KO mice will be sacrificed by cervical dislocation, spinal rapidly removed, dissociated from fat tissue and placed in cold isolation buffer (41). Tissue will be minced, homogenized and centrifuged until mitochondria are isolated and incubated in the absence of hydrogen peroxide (23).
To measure hydrogen peroxide activity from isolated mitochondria, mitochondria will be diluted in buffer in the presence of sodium citrate and rotenone. At appropriate points, an aliquot will be transferred into an assay mixture containing 50mM KHPO and 1microM DCFDA. Florescence readings at 485 nm will be taken and plotted on a curve.
Prediction: We predict that florescence readings from conditional KO mice will be significantly higher than readings obtained from WT mice indicating a high level of hydrogen peroxide output in conditional KO mice. An insignificant difference in H2O2 output between conditional KO and WT mice will suggest that H2O2 is not the main pathology of frataxin deficiency leading to FRDA. While this may be possible, it does not exclude the possibility of oxidative stress as a main pathological feature and cause of FRDA, as other sources of oxidation are present in a cell.
2. Investigate the role of hydrogen peroxide in catalase deficient and catalase augmented FRDA conditional knockout and WT mice
Rationale: The finding that hydrogen peroxide scavenging in Drosophila restores lifespan and reduces symptomatology in frataxin deficient flies is yet to be replicated using a mammalian model of FRDA. Therefore, we seek to replicate the methodology used by Anderson et al. (2007) using conditional knockout mice as our model organism. Consistent with the hypothesis that hydrogen peroxide plays a critical role in FRDA pathogenesis, we believe that catalase over expression will reduce symptoms and increase the lifespan of our conditional KO mice. Conversely, silencing the catalase gene will worsen the symptoms and lifespan of our mice model.
Design and Method
Effects of catalase overexpression in conditional KO mice: To determine the effects of lessening the concentration of intracellular hydrogen peroxide in our FRDA mouse model, mice overexpressing catalase will be crossed with frataxin conditional KO mice. Real Time RT-PCR and Western Blot analysis will be used to determine progeny that exhibit both catalase over expression and neuronal frataxin knockout. Transgenic catalase overexpressing mice (Tg(CAT)(+/+)) are provided as described in ref. 43, and are generated by using human DNA fragment containing the human CAT gene. Selected progeny will be subject to the same hydrogen peroxide assays, movement tasks, and physical analysis as described in Aim 1.
Prediction: We predict that conditional KO mice expressing human catalase will exhibit fewer signs of FRDA pathology as determined by observation of physical characteristics such as gait, weight, posture, and movement. Moreover, we expect augmented conditional KO mice to exhibit a longer lifespan compared to conditional KO mice without human catalase, consistent with the results of Anderson et al. (26) in a Drosophila model. We also expect a significantly lower output of hydrogen peroxide in chimeras with respect to WT mice. The absence of any significant improvement in physical as well as intracellular hydrogen peroxide accumulation could suggest that another source of oxidative stress other than hydrogen peroxide may be responsible for the underlying pathogenesis of FRDA.
Effects of catalase deficiency in frataxin conditional knockout mice:
To provide further evidence that indeed hydrogen peroxide is crucial to the pathogenesis of FRDA, we will determine the effects of catalase KO in our frataxin conditional KO mice. Previous research has shown that catalase KO mice do not exhibit any serious defects and function as healthy mice (42). Consistent with our methodology described above, transgenic catalase KO mice [Tg(CAT)(-/-)], generated as described in (42), will be crossed with our previously developed conditional knockout mice. Progeny exhibiting both catalase deficiency and frataxin deficiency in neuronal cells will be isolated and used for data analysis. Selected progeny will be assessed as done previously in Aims 1 & 2 to characterize physical as well as intracellular activity of hydrogen peroxide.
Prediction: We believe that hydrogen peroxide is critical to the pathology of FRDA. Therefore, we predict that reducing the rate of hydrogen peroxide degradation by knocking down the catalase gene will aggravate the symptoms of FRDA and further reduce the lifespan of our conditional KO mice. Moreover, we expect that tissue extracted from selected progeny will indicate an above average output of hydrogen peroxide. A nonsignificant change in the physical condition and lifespan of conditional KO (CAT(-/-)) mice could suggest that hydrogen peroxide is not the main source of FRDA symptomatology.
3. Characterize the role of aconitase and other iron metabolism regulating genes in FRDA conditional knockout and WT mice
Rational: Frataxin deficiency has been found to cause both mitochondrial iron-loading and H2O2 toxicity in FRDA patients’ cells, and catalase has been shown to rescue FRDA cells in Drosophila (5, 16, 29). If catalase over expression is rescuing cells with mitochondrial iron-loading, then we believe that it should be returning the expression of down regulated genes involved in mitochondrial iron synthesis pathways back to or near healthy levels (5). Thus, we will compare the FRAT-KO with WT mice to compare gene expression levels. We will specifically examine Alad, Hmbs, Uros, Cpox, Fech, Nfs1, Iscu1/2, Hfe2, and Ftmt. In addition, we will also measure ferredoxin reductase (Fdxr) because Fdxr is believed to act as a compensatory mechanism for mitochondrial regulation in the case of a frataxin deficiency (30). Since aconitase is sensitive to oxidative stress from superoxides, its activity will also be observed (31, 32).
Design and Method
Investigation of the expression of iron metabolism regulating genes in FRAT-KO mice PNS cells over expressing catalase:
The conditional frataxin knockout mice will be created using methods described in Aim 1, and catalase will be both over and under expressed in FRAT-KO and WT mice using the methods from Aim 2. Mice will be sacrificed at 4 and 10 weeks to see alterations in gene expression over time, which was found to be significant in a previous study (27). The mitochondrion of the cells will be isolated through the use of differential centrifugation (23). Gene expression will then be analyzed in the FRAT-KO and WT mice with over, under, and normally expressed catalase activity at both 4 and 10 weeks. This creates 12 groups, including WT mice with over expressed catalase activity as the positive control, FRAT-KO mice with under expressed catalase activity as the negative control, as well as several experimental groups and two more control groups. The expression of the iron metabolism regulating genes (Alad, Hmbs, Uros, Cpox, Fech, Nfs1, Iscu1/2, Hfe2, and Ftmt) will be measured using several techniques: a western blot to analyze protein expression, RT-PCR (which measures mRNA expression) to measure fold change, and microarray analysis, which will give a principal component plot of overall expression. This will allow us to look at the expression of all the expressed genes to see what other differences there are between the mice, other than the 10 genes we know to change (30, 27).
Prediction: If catalase over expression rescues the cells’ mitochondrial iron metabolism, then we expect to see gene regulation to return to normal in the FRAT-KO mice with over expressed catalase. Since FRAT-KO mice have been found to have Alad, Hmbs, Uros, Cpox, Fech, Nfs1, Iscu1/2, Hfe2 and Ftmt down-regulated and Fdxr up-regulated, we expect that their regulation will return to normal compared to the WT mice in both the western blot and the RT-PCR (27). We also expect that the over expression of catalase in WT mice will cause the up-regulation of iron regulating genes and the down-regulation of Fdxr in both tests (27). We also expect the under expression of catalase to have the opposite effect in the regulation of genes. If over expression of catalase has no effect on iron metabolism regulating hormones, which is our alternative hypothesis, then we expect the western blot and RT-PCR to resemble that of regular FRAT-KO and WT mice with normal expression of catalase. If our hypothesis is correct, then we expect the microarray analysis to show that the principal component plot of overall expression in FRAT-KO and WT mice at 4 and 10 weeks with over expressed catalase to look the same. If the hypothesis is incorrect, there will be noticeable differences (27).
Characterization of aconitase activity in FRAT-KO mice PNS cells over expressing catalase:
To assay aconitase activity, mitochondria will be diluted in potassium hydrogen phosphate (K2HPO4) containing triton. Aconitase activity in WT and FRAT-KO mice will be assayed as the rate of NADP+ reduction (at 340nm) by isocitrate dehydrogenase when sodium citrate, NADP+, and isocitrate dehydrogenase are added (23). MS745 aconitase activity screening protocol will be used to measure aconitase activity (35). Spectrophotometry will be used to measure absorbance at 0 and 30 minutes of the aconitase assays; the difference in absorbance will be an indication of aconitase activity, specifically the rate of absorbance (mOD/min) (34, 35). This will be done using an assay kit that we will purchase from Mitosciences (35).
Prediction: If our hypothesis is correct, we expect to see a decrease in aconitase activity in FRAT-KO mice with no catalase over expression and WT mice with catalase under expression; thus, there will be a smaller difference in the absorbencies of samples with decreased aconitase activity. We also expect this to be reversed, have the catalase rescue the cells and thus increase the difference in absorbencies, in the WT mice and the FRAT-KO and WT with the over expression of catalase. If the alternative hypothesis is correct, that aconitase activity is not affected by a frataxin deficiency and catalase has no effect on aconitase activity, then the difference in absorbencies will not be affected by our manipulations.
References
1. Bredesen DE, Rao RV, Mehlen P (October 2006). “Cell death in the nervous system”. Nature 443 (7113): 796–802. doi:10.1038/nature05293. PMID 17051206
2. DiMauro S, Schon EA (2008). “Mitochondrial disorders in the nervous system”. Annual Review of Neuroscience 31: 91–123. doi:10.1146/annurev.neuro.30.051606.094302. PMID 18333761
3. Delatycki MB, Williamson R, Forrest SM. Friedreich ataxia: an overview. J Med Genet 2000;37:1. [PubMed: 10633128]
4. Pandolfo M, Pastore A (2009) The pathogenesis of Friedreich ataxia and the structure and function of frataxin. J Neurol 256:9-17.
5. Babcock M, et al. (1997) Regulation of mitochondrial iron accumulation by Yfh1p, a putative homolog of frataxin. Science 276:1709-1712.
6. Becker EM, Greer JM, Ponka P, Richardson DR (2002) Erythroid differentiation and protoporphyrin IX down-regulate frataxin expression in Friend cells: Characterization of frataxin expression compared to molecues involved in iron metabolism and hemoglobinization. Blood 99:3813-3822.
7. Bencze KZ, et al. (2007) Human frataxin: iron and ferrochelatase binding surface. Chem Commun 14:1798-1800.
8. Campuzano V, et al. (1996) Friedreich’s Ataxia: Autosomal Recessive Disease Caused by an Intronic GAA Triplet Repeat Expansion. Science 271:1423-1426.
9. Sakamoto N, Oshima K, Montermini L, Pandolfo M, Wells RD (2001) Sticky DNA, a self-associated complex formed at long GAATTC repeats in intron 1 of the frataxin gene, inhibits transcription. J Biol Chem 276: 27171-27177.
10. Bidichandani SI, Ashizawa T, Patel PI. The GAA triplet-repeat expansion in Friedreich ataxia interferes with transcription and may be associated with an unusual DNA structure. Am J Hum Genet 1998;62:111. [PubMed: 9443873]
11. Ohshima K, Montermini L, Wells RD, Pandolfo M. Inhibitory effects of expanded GAA.TTC triplet repeats from intron I of the Friedreich ataxia gene on transcription and replication in vivo. J Biol Chem 1998;273:14588. [PubMed: 9603975]
12. Pandolfo M. Iron metabolism and mitochondrial abnormalities in Friedreich ataxia. Blood Cells Mol Dis 2002;29:536. [PubMed: 12547248]
13. Lesuisse E, et al. (2003) Iron use for haeme synthesis is under control of the yeast frataxin homologue (Yfh1). Human Molecular Genetics 12:879-889.
14. Rotig A, et al. (1997) Aconitase and mitochondrial iron-sulpher protein deficiency in Friedreich ataxia. Nat Genet 17:215-217.
15. Wilson RB. (2006). Iron dysregulation in Friedreich ataxia. Seminars in Pediatric Neurology. 13(3), 166-75.
16. Armstrong JS, Khdour O, & Hecht SM. (2010). Does oxidative stress contribute to the pathology of Friedreich’s ataxia? A radical question. The FASEB Journal : Official Publication of the Federation of American Societies for Experimental Biology. 24(7), 2152-63.
17. Puccio H, et al. (2001) Mouse models for Friedreich Ataxia exhibit cardiomyopathy, sensory nerve defect and Fe-S enzyme deficiency followed by intramitochondiral iron deposits. Nat Genet 27181-186.
18. Lee, K., Iijima-Ando, K., Iijima, K., Lee, W., Lee, J., Yu, K., et al. (2009). JNK/FOXO-mediated Neuronal Expression of Fly Homologue of Peroxiredoxin II Reduces Oxidative Stress and Extends Life Span. J Biol Chem, 284(43), 29454-29461. doi:10.1074/jbc.M109.028027.
19. Li H, Gakh O, Smith DY 4th, & Isaya G. (2009). Oligomeric yeast frataxin drives assembly of core machinery for mitochondrial iron-sulfur cluster synthesis. J Biol Chem. 284(33), 21971-80.
20. Park S, Gakh O, Mooney SM, & Isaya G. (2002). The ferroxidase activity of yeast frataxin. J Biol Chem. 277(41), 38589-95.
21. Chen OS, Hemenway S, Kaplan J. Inhibition of Fe-S cluster biosynthesis decreases mitochondrial iron export: evidence that Yfh1p affects Fe-S cluster synthesis. Proc Natl Acad Sci U S A 2002;99:12321. [PubMed: 12221295.
22. Beinert H, Kennedy MC, Stout CD. Aconitase as ironminus signsulfur protein, enzyme, and ironregulatory protein. Chem Rev 1996;96:2335. [PubMed: 11848830]
23. Bulteau AL, Ikeda-Saito M, Szweda LI. Redox-dependent modulation of aconitase activity in intact mitochondria. Biochemistry 2003;42:14846. [PubMed: 14674759]
25. Cavadini P, Gellera C, Patel PI, Isaya G. Human frataxin maintains mitochondrial iron homeostasis in Saccharomyces cerevisiae. Hum Mol Genet 2000;9:2523. [PubMed: 11030757]
26. Karthikeyan G, Lewis LK, Resnick MA. The mitochondrial protein frataxin prevents nuclear damage. Hum Mol Genet 2002;11:1351. [PubMed: 12019217]
27. Anderson PR, Kirby K, Hilliker AJ, & Phillips JP. (2008). Hydrogen peroxide scavenging rescues frataxin deficiency in a Drosophila model of Friedreich’s ataxia. PNAS 105(2):611-616.
28. Huang LM, Becker EM, Whitnall M, Rahmanto YS, Ponka R, Richardson DR (2009) Elucidation of the mechanism of mitochondrial iron loading in friedreich’s ataxia by analysis of a mouse mutant. PNAS 106:16381-16386.
29. Bulteau AL, Lundberg KC, Ikeda-Saito M, Isaya G, Szweda LI. Reversible redox-dependent modulation of mitochondrial aconitase and proteolytic activity during in vivo cardiac ischemia/reperfusion. Proc Natl Acad Sci U S A 2005;102:5987. [PubMed: 15840721]
30. Anderson PR, Kirby K, Hilliker AJ, & Phillips JP. (2005). RNAi-mediated suppression of the mitochondrial iron chaperone, frataxin, in Drosophila. Human Molecular Genetics. 14(22), 3397-405.
31. Li J, Saxena S, Pain D, Dancis A (2001) Adrenodoxin reductase homolog (Arh1p) of yeast mitochondira required for iron homeostasis. J Biol Chem 276:1503-1509.
32. Schultz JB, Dehmer T, Schols L, Mende H, Hardt C, Vorgerd M, Burk K, Matson W, Dichgans J, Beal MF. Oxidative stress in patients with Friedreich’s ataxia. Neurology 2000;55:1719. [PubMed: 11113228]
33. Vazquez-Manrique RP, Gonzalez-Cabo P, Ros S, Aziz H, Baylis HA, Palau F. Reduction of Caenorhabditis elegans frataxin increases sensitivity to oxidative stress, reduces lifespan, and causes lethality in a mitochondrial complex II mutant. Faseb J 2006;20:172. [PubMed: 16293572]
34. Alter O, Brown PO, Botstein D (2000) Singular value decomposition for genome-wide expression data processing and modeling. PNAS USA 97:10101-10106.
35. Narang S, Seawright JA, Willis NL (1987) Genetic mapping, distribution, and properties of an aconitase isozyme in Anopheles albimanus (Diptera: Culcidae). Biochem Genet 25(1-2):67-77. [PubMed: 3579868]
36. Mitoscience. Aconitase enzyme activity microplate assay kit. Retrieved from http://www.mitosciences.com/PDF/aconitase_enzyme_activity_microplate_assay_protocol.
37. Kaplan J. (1999). Friedreich’s ataxia is a mitochondrial disorder. Proceedings of the National Academy of Sciences of the United States of America. 96(20), 10948-9
38. Pandolfo M. (2008). Friedreich ataxia. Archives of Neurology. 65(10), 1296-303
39. Navarro JA, Ohmann E, Sanchez D, Botella JA, Liebisch G, Moltó MD, Ganfornina MD, Schmitz G, & Schneuwly S. (2010). Altered lipid metabolism in a Drosophila model of Friedreich’s ataxia. Human Molecular Genetics. 19(14), 2828-40.
40. Lesuisse E, Santos R, Matzanke BF, Knight SA, Camadro JM, & Dancis A. (2003). Iron use for haeme synthesis is under control of the yeast frataxin homologue (Yfh1). Hum Mol Genet. 12(8), 879-89.
41. Li K, Besse EK, Ha D, Kovtunovych G, & Rouault TA. (2008). Iron-dependent regulation of frataxin expression: implications for treatment of Friedreich ataxia. Hum Mol Genet. 17(15), 2265-73
42. Schriner SE, Linford NJ, Martin GM, Treuting P, Ogburn CE, Emond M, Coskun PE, Ladiges W, Wolf N, Van Remmen H, Wallace DC, & Rabinovitch PS. (2005). Extension of murine life span by overexpression of catalase targeted to mitochondria. Science (New York, N.Y.). 308(5730), 1909-11.
43. Ho YS, Xiong Y, Ma W, Spector A, & Ho DS. (2004). Mice lacking catalase develop normally but show differential sensitivity to oxidant tissue injury. J Biol Chem. 279(31), 32804-12.
44. Chen X, Liang H, Van Remmen H, Vijg J, & Richardson A. (2004). Catalase transgenic mice: characterization and sensitivity to oxidative stress. Archives of Biochemistry and Biophysics. 422(2), 197-210.