Two Studies on Parkinson’s Disease Protein, α-Synuclein: Amino Acid Determinants and Truncations
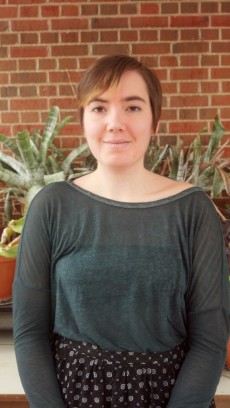
Katrina J. Campbell
Department of Biology & Neuroscience
Lake Forest College
Lake Forest, Illinois 60045
Download PDF
Abstract
Parkinson’s disease (PD) is a disease in which neurons in the substantia nigra die. Within these neurons are Lewy bodies, proteinatious aggregates composed primarily of α-synuclein. Two important questions in PD research are these: are some amino acids more important in the properties of α-synuclein; how are naturally-occurring C-terminal truncations to α-synuclein relevant to pathology? My senior thesis explores both questions. To evaluate the importance of specific amino acids, I made point mutations on implicated amino acids: D2, A76, V77, Q79, and E83. I hypothesized that these mutations would either diminish or enhance the pathological properties of α-synuclein. I found that these amino acids are important for t he properties of α-synuclein. To study the relevance of truncations, I recreated four naturally occurring truncations. I hypothesized that t h e truncations would result in aggregations because of the solubility properties of the C-terminus. Truncation resulted in less membrane binding and cytoplasmic punctate foci.
Introduction
Proteins
Throughout the three kingdoms of life, organismal diversity is evident. Some organisms, such as humans have trillions of highly specialized cells while others, like yeast, are simple single-celled organisms. Despite this diversity, all organisms consist of essentially the same ingredients: sugars, DNA, RNA, fats, proteins, and other small molecules (Cooper, 2000). Of all these components, proteins are the most diverse. Proteins range widely in size, charge, structure, and function. The hundreds of thousands of specific proteins in each cell give rise to the enormous diversity between cells (Alberts et al., 2009).
Proteins do the majority of work within a cell. They perform many jobs such as providing structural support, transporting molecules, sending messages within and between cells, and aiding in chemical reactions (Alberts et al., 2009). A protein that is the same phenotype as the general population is said to be wild-type (WT) and will generally perform its usual function within the cell. There are ways in which proteins can lose their function through mutation, concentration changes, or misfolding (Reynaud, 2010). If the DNA sequence that encodes a protein becomes mutated, this may cause a change in the amino acid sequence that comprises the protein resulting in a mutant protein. The promoter region of the same gene can become mutated so less or more of the protein is made. A protein can also misfold. This becomes more likely as the concentration of the protein increases. The likelihood that any one protein will misfold is small but the likelihood that any protein will misfold increases as the concentration of protein increases. Other factors such as cellular stress and chaperone proteins also impact protein folding. Protein misfolding can also be caused by mutations that changes key amino acids of the protein (Reynaud, 2010).
If a protein is mutated, it can have one of three outcomes. The first option, and the most common outcome, is that the protein continues to carry out its normal function. In this case, the properties of the mutated amino acid are not different enough to result in alternate folding. These are called neutral mutations. The mutant amino acid in this case does not generally occupy an important binding site or a key site for folding so the protein can continue to function properly. Because these mutations are not under selective pressure, they can be helpful in understanding the evolutionary history or an organism (Kimura, 1968). A less likely outcome of a mutation is that the resulting protein is beneficial in some way to the organism, conferring an advantage over other organisms and may reproduce more, causing the mutation to be perpetuated. An example of this is the point mutation in the hemoglobin gene that causes sickle cell anemia. This mutation confers an advantage when heterozygous by protecting against malaria (Allison, 1964). The third possibility is that the change in protein shape or function causes disease. This can occur through a protein’s gain of a toxic function or loss of a vital function. An example of a gain of toxic function is if a protein misfolds and forms toxic aggregates such as beta-amyloid that form plaques in Alzheimer’s disease (Masters et al., 1985). In the case of cystic fibrosis, a chloride channel is mutated so it can no longer perform its normal function (Rommens et al., 1989). If a protein provides a vital function for the cell and can no longer function properly, the cell will die.This loss of function or gain of toxic function of a protein can lead to disease. Proteins are the culprit for many common diseases today.
Neurodegenerative Diseases
Protein-misfolding diseases can happen throughout the body. An example of this is the chloride ion channel, CTFR, which causes Cystic Fibrosis, a disease that affects the lungs and other tissues (Rommens et al., 1989). Neurodegenerative diseases are subset of protein-misfolding diseases that affect neurons or their supporting cells. Each neurodegenerative disease affects a specific subset of cells (Figure 1) such as: the cerebral cortex and hippocampus in Alzheimer’s disease (Khachaturian, 1985), the striatum in Huntington’s disease (Lange, 1976), or the substantia nigra in Parkinson’s disease (Marsden, 1990). In each illness, a different protein has aggregated and come out of solution (Figure 1): beta amyloid and tau in Alzheimer’s disease (Masters et al., 1985; Glenner et al., 1988; Wischik et al., 1988), Huntington in Huntington’s disease (The Huntington’s Disease Collaborative Research Group, 1993), and α-synuclein in Synucleinopathies (Spillantini et al., 1997; Spillantini et al., 1988; Baba et al., 1978).
Synucleinopathies are a set of neurodegenerative diseases marked by fibrillary aggregates of α-synuclein in the cytoplasm of both neurons and glia (Trojanowske & Lee, 2003). Synucleinopathies include: dementia with Lewy bodies, pure autonomic failure, multiple system atrophy, and Parkinson’s disease. Lewy bodies are the aggregation of α-synuclein within the cytoplasm of neurons and occur in dementia with Lewy bodies, pure autonomic failure, and Parkinson’s disease. In multiple system atrophy, α-synuclein forms similar aggregations in oligodendrocytes (Martí, Tolosa, & Campdelacreu,2003). Mythesisfocuseson Parkinson’s disease.
Parkinson’s disease
Parkinson’s disease (PD) affects seven to ten million people worldwide, making it the second most prevalent neurodegenerative disease after Alzheimer disease (Zhang & Román, 1993). PD primarily affects the substantia nigra (Marsden, 1990; Figure 1), a part of the basal ganglia, the area of the brain that controls the speed and strength of movement (Marsden & Fahn, 1982).
Parkinson’s disease is characterized by its cardinal symptoms: tremor at rest, brady/hypo/akynesia (an overall slowing down, scaling down, or loss of movement), freezing of gait, flexed posture, rigidity, and loss of postural reflexes. In order for a patient to be diagnosed with PD, they must exhibit at least two of these symptoms with at least one of them being tremor at rest or bradykinesia. Tremor at rest, bradykinesia, and rigidity generally appear early in the course of the disease. The later symptoms include freezing of gait, flexed posture, and loss of postural reflexes (Nass & Przedborski., 2008). As the disease progresses, movements become slower as in bradykinesea and may stop completely or scale down in size as in akinesea and hypokinesea, respectively. Tremor at rest, the other hallmark symptom of PD, is an unwilling oscillation of a body part when at rest. Tremors often begin on one side of the body and move to the other side as the disease progresses (Hughes et al., 1993). Although PD is primarily diagnosed by its motor symptoms, it also has a myriad of accompanying non-motor symptoms. Some of these symptoms include: depression, insomnia, fatigue, dry eyes/mouth, weight loss, and nausea (Chaudhuri & Quinn, 2006). These symptoms can present at any stage of PD progression and may be present even before motor symptoms (Koller, 1992).
Figure 1: The diversity of synucleinopathies Presented is a midsagittal view of the brain with an overview of where different synucleinopathies exist. Dementia with Lewy Bodies affects the cortex and is shown in blue. Multiple system atrophy affects many areas, including the caudate nucleus, putamen, and substantia nigra and is shown in purple. Pure autonomic failure affects the intermediolateral column of the spinal cord and is in teal. Parkinson's disease is shown in orange and affects the substantia nigra. Each disease has a specific pattern of α-synuclein deposits. The images were adapted from the following sites: Brain image- http://www.anatomybox.com/brain-sagittal-view/, Dementia with Lewy Bodies- http://www.medicinenet.com/image-collection/lewy_body_dementia_picture/picture.htm, Multiple System Atrophy- http://en.wikipedia.org/wiki/Multiple_system_atrophy, Pure Autonomic Failure- http://www.neurology.org/content/56/7/980/F1.expansion.html, Parkinson's Disease- http://www.nature.com/nrn/journal/v4/n1/images/nrn1007-f1.jpg
PD affects the substantia nigra of the midbrain (Marsden, 1990). These dopaminergic neurons produce neuromelanin, giving the substantia nigra a dark color and its name which translates to ‘dark substance’ (Adler, 1939). During the progression of PD, these neurons selectively die (Fearnley & Lees, 1991). The substantia nigra is a part of the basal ganglia which also include the caudate nucleus and putamen, the globus pallidus, and the subthalamic nucleus. Through a series of inhibitory and excitatory connections, the basal ganglia allow desired movements and repress undesired movements (Squire et al., 2012). When the substantia nigra degenerates, it releases less dopamine into this system. Because of the loss of these inputs, the overall effect is the slowing down of desired movement and the increase in undesired movement producing hypokinesia and tremor at rest, respectively.
PD can onset at any age, but the risk increases with age into late adulthood. The average onset is about sixty years (Nass & Przedborski, 2008) and about 4% of people who will develop PD are diagnosed by age fifty. Males are about fifty percent more likely to develop PD than women. The mechanism for this difference is unknown, but some researchers speculate this might be due to physiological differences between the sexes or to factors to which men are more often exposed (Wooten et al., 2004). For instance, men are two times more likely than women to have a traumatic head injury (Kraus & MacArthur, 1996) and males are also more likely to be exposed to toxic chemicals during traditionally masculine work such as farming. Alternatively, females may be at a lower risk due to an increased amount of estrogen. Hormone replacement therapies have been shown to protect against PD in postmenopausal women (Currie et al., 2004). Studies have shown that estrogen is as an antioxidant against oxidative stress and may have neuroprotective properties (Nestrosa et al., 1998).
Parkinson’s disease can be acquired two ways: genetically and sporadically. Sporadic PD is the more common form of PD and an individual’s risk factor is influenced by environmental factors and recessive genetics (Nass & Przedborski, 2008). Rural living increases PD risk as well as a close association with well water and farming. Pesticides like those used in farming can cause oxidative stress within cells and have been linked to an increased risk of PD (Priyadarshi et al., 2001; Abdollahi et al., 2004). Common pesticides directly increase the rate of α-synuclein fibril formation (Uversky et al., 2001). Head trauma also increases the risk of PD development, even in cases where the head injury occurred decades before PD diagnosis (Goldman et al., 2006).
PD can be caused by genetic mutations in a variety of genes and is passed through families. This type of PD is often referred to as familial PD because of this pattern of inheritance. Familial PD only accounts for about ten percent of PD cases. Individuals with PD-affected family members have a three-to-four fold increase in disease risk than those without a family history of PD (Kurza et al., 2003). Although familial PD is much rarer than the sporadic form, it provides us with valuable information about the process of PD acquisition. Familial Parkinson’s disease can be caused by a mutation in multiple genes. One such gene is SNCA, the gene that encodes of α- synuclein. Individuals who have a duplication or triplication of this gene make double or triple the amount of α- synuclein and generally get a more aggressive form of PD with an early age of onset (Chartier-Harlan et al., 2003). Single amino acid mutations in α- synuclein can also cause familial Parkinson’s disease. The first of these mutations found was the A53T mutation (Polymeropoulos et al., 1997). Later, researchers discovered the A30P (Kruger et al., 1998) and E46K (Zarranz et al., 2004) mutations as a cause of familial PD. Recently, two more mutations, H50Q (Appel-Cresswell et al., 2013), and G51D (Lesage et al., 2013) were also discovered. The direct relation between PD and α- synuclein dosage and mutations very strongly implies that α-synuclein is crucial to PD onset.
As illustrated by figure 2, Additional genes that can cause familial PD include: Park9 (Najim et al., 2004), parkin (Kitada et al., 1998), UCH-L1 (Maraganore et el., 2004), PINK (Valente et al., 2004), DJ-1 (Bonifati et al., 2002), and LRRK2 (Zimprich et al., 2004). Parkin (Shimura et al., 2000) and UCH-L1 (Leroy et al., 1998) are a part of a system that marks proteins for proteasome degradation. DJ-1, Pink1, and Parkin form an ubiquitin ligase to degrade parkin and parkin substrates. Impaired function of this complex can result in a toxic build-up of parkin and its substrates (Xiong et al., 2009). The LRRK2 gene encodes a protein that assists with intracellular signaling associated with chaperone responses, cytoskeleton maintenance, and phosphorylation and kinase activity (Dӓche et al., 2007). If the LRRK2 gene is mutated, this function may be lost, causing problems within the cell, but the mutation may also increase the kinase activity resulting in a gain of function toxicity (Greggio et al., 2006). In a healthy cell, Park9 mediates manganese transport, protecting the cell from toxicity (Gitler et al., 2009). LRRK2, Pink1, and Parkin all mediate mitochondrial function when working properly and their impairment can lead to mitochondrial damage and oxidative stress (Norris & Giasson, 2005). The PINK and DJ-1 proteins serve as protective agents to mitochondria during cellular stress (Deng et al., 2008; Yang et al., 2005). Many of the proteins that can cause PD are either involved with protein degradation or protecting the cell from stressors (Figure 2) which are closely linked to the factors for developing sporadic PD. These proteins, like any molecule, exist in a balance within a cell. If this balance is tipped in one direction or another, disease may result. α-Synuclein exists in a balance of production and degradation within cells. Disease occurs if more α- synuclein is made as in a duplicated or triplicated gene, or less is degraded, like in cells with mutant parkin or UCH-L1 proteins.
The role of α-synuclein in familial and sporadic PD along with the composition of Lewy Bodies has led many researchers in the field of Parkinson’s disease to focus on α- synuclein and its role in the disease. α-synuclein is closely related to β- and γ- synuclein. The three proteins are 55-62% conserved, have similar domain organization (Lavedan, 1998), and are small and natively unfolded (Clayton & George, 1998). α-Synuclein is 140 amino acids long with six exons and five introns (Tan et al., 204). It consists of three domains, the N-terminus, M domain, and the C-terminus (Goedert, 2001).
α-, β-, and γ-synuclein can form α- helical structures on the N-terminus when bound to a membrane (Davidson et al., 1998). The N-terminus (amino acids 1-60) consists of two lipid-binding α helices (Sreeganga et al., 2003). α-Synuclein in its helical form is much more prone to aggregation than in its unfolded form (Chiti et al., 2003). Moreover, this helical form can act as a seed for other α-synuclein proteins, causing even more aggregation (Lee et al., 2002). The NAC domain (amino acids 61-95) is found in α- but not β- or γ-synuclein (Biere, 2000). Neither β- nor γ-synuclein form fibrils in solution like α-synuclein does (Biere, 2000). The NAC domain is hydrophobic and unstructured and is necessary and sufficient for α-synuclein fibrillization (Bodes et al., 2001; Giasson et al., 2001). The C-terminus (amino acids 95-140) is acidic with glutamate residues and resists aggregation (Clayton & George, 1999). The full function of α-synuclein in a healthy cell is unknown, but it is known to inhibit PLD2, a membrane enzyme that plays a role in cell secretion (Jenco et al., 1998). α-Synuclein is located in the presynaptic nerve terminal of neurons (Hsu et al., 1998). Its location and interaction with PLD2 implies that α-synuclein may play a role in secretion.
Figure 2: Many genes can cause Parkinson's disease This is a diagram of how other genes other than SNCA can cause PD. Black arrows represent normal function. Red arrows denote toxic, gain of function properties that occur under stress or when mutated. Red bars through black arrows are where normal function is lost when under stress or when mutated. LRRK2 may regulate mitochondrial function, which is lost when Parkinson's disease occurs. LRRK2 also undergoes a kinase-mediated gain of function that results in cellular toxicity. DJ1, PINK1, and PARKIN are a part of the PDD complex which helps protein degradation. If DJ-1, PINK1, or PARKIN are mutated and cannot form the complex, protein degradation is impaired, resulting in a toxic buildup of misfolded proteins. During impaired protein degradation PARKIN also produces substrates that are toxic to the mitochondria. If UCHL1 is not functioning properly, protein degradation is impaired. PARK9 protects cells from manganese toxicity through assisting with manganese transport. When this function is lost, manganese toxicity increases.
In a diseased cell, α-synuclein may play many roles (Figure 3). α-Synuclein blocks the secretion of newly formed proteins through inhibition of the ER-Golgi pathway (Cooper et al., 2006). As α-synuclein begins to accumulate and misfold, it inhibits autophagy and the proteasome which leads to less overall protein degradation (Martinez-Vicente et al., 2008). α-Synuclein is degraded through autophagy (Cuervo et al., 2004) and the proteasome (Tanaka et al., 2001), so it blocks the processes by which it is degraded, exacerbating the problem. α-Synuclein also disrupts endocytosis (Nemani et al., 2010). It also disrupts microtubules through interactions with tau (Sousa et al., 2009), a protein which associates with and stabilizes microtubules (Lee et al., 2001). α-Synuclein promotes tau fibrillization which causes less tau-microtubule association and leads to destabilization (Giasson et al., 2003). Actin fibers are destabilized by α-synuclein which increases the rate of depolymerization and decreases the rate of actin polymerization (Sousa et al., 2009).
Even though α-synuclein is found throughout the brain, the details as to why it selectively kills dopaminergic neurons are still unclear (Hirsch et al., 1988). Dopaminergic neurons are already under oxidative stress due to high levels of iron and dopamine oxidation (Lotharius et al., 2002) and α-Synuclein adds stress to this system (Perez & Hastings, 2004). α-Synuclein protofibrils have also been shown to be toxic to cells (Lashuel et al., 2002). Dopamine binds to α-synuclein in such a way that allows protofibril formation, but not full fibrils (Rochet et al., 2004). This increases the overall amount of α-synuclein protofibils within the cell. These mechanisms may work in conjunction to result in dopamine-specific cell death.
Once α-synuclein begins to aggregate, Lewy bodies, large conglomerates of the protein are formed (Pollanen et al., 1993; Figures 1 & 3.5). While one might claim that Lewy bodies are the toxic species in PD, this topic is a point of dissent within the field. Lewy bodies have not been proven to be either good or bad for the affected cells (Da Costa et al., 2000; Tabrizi et al., 2000). Some studies suggest that smaller α-synuclein oligomers and protofibrils are much more toxic and Lewy bodies develop and sequester this more toxic form (Goldberg & Lansbury, 2000).
α-Synuclein is a heavily modified protein. It is phosphorylated at S87, S129, Y125, Y133, and Y136 (Fujiwara et al., 2002; Anderson et al., 2006). The effect of phosphorylation on toxicity is a complex issue. α-synuclein phosphorylation at S129 in Drosophila produces toxicity (Chen & Feany, 2005) whereas phosphorylation at S129 confers a protective effect in rat models (Gorbatyuk et al., 2008; Chen et al., 2009). α- Synuclein is also nitrated at T39, T125, T133, and T136 (Clayton & George, 1998).
Nitration increases the propensity of α-synuclein to form fibers and nitration on T39 especially reduces the ability of α-synuclein to bind membranes much like the familial mutation on the nearby A30 prevents membrane binding (Hodara et al., 2004). Two more sites that undergo modifications have been discovered more recently. K96 and K102 are modified through covalent bonds to the small ubiquitin-like modifier protein, more commonly known as SUMO (Dorval & Fraser, 2006). SUMO mediates protein localizations, interactions, and even degradation through competition with ubiquitin (Dohmen, 2004). SUMO prevents α-synuclein from binding to itself, reducing aggregation (Krumova et al., 2011).
In addition to covalently attached chemical groups, α-synuclein is found in smaller forms created by either alternate splicing or protease cleavage. Naturally- occurring splice variants have recently been discovered (Beyer et al., 2004; Campion et al., 1995). One variant is 112 amino acids long and is missing exon five, amino acids 103-130. Another variant is 126 amino acids total and is missing exon three between amino acids 41-53 (Beyer et al., 2006). A splice variant also exists that is missing both exons three and five and is 98 amino acids long (Beyer et al., 2008). Along with these modifications, truncations formed by protease cleavage have been found both in healthy and Parkinson’s disease affected patients (Liu et al., 2005; Li et al., 2005; Lewis et al., 2010).
On the topic of how α-synuclein affects PD, there are several big questions that researchers are trying to answer. These questions include: How does α-synuclein imbalance lead to Parkinson’s disease? What causes α-synuclein to become toxic to the cell? How do modifications play a role in pathology? How does α-synuclein accumulation get out of balance in disease? How do natural variations of α-synuclein affect its pathological properties? Do certain amino acids have a larger impact on the properties of α-synuclein? From these questions, I chose to focus on if certain amino acids have a larger impact on the properties of α-synuclein and how the natural variations of α-synuclein affect its pathological properties. I will finish my introduction by discussing in more detail my questions and my model.